Difference between revisions of "Environmental Impact of Stressors (Sustainability Assessment)"
Line 310: | Line 310: | ||
but it could result in follow-up actions.”</blockquote> | but it could result in follow-up actions.”</blockquote> | ||
Although the dose limits, expressed as an annual effective dose to the representative person, are generally | Although the dose limits, expressed as an annual effective dose to the representative person, are generally | ||
− | set at the level of 1 mSv, dose constraints are quite different in different countries (see Table | + | set at the level of 1 mSv, dose constraints are quite different in different countries (see Table 12, in Section II.2.3). |
It should be emphasized that there are no constraints for the release of individual radionuclides. There is | It should be emphasized that there are no constraints for the release of individual radionuclides. There is | ||
also no international recommendation on the limits for concentrations of radionuclides in the environment. On the | also no international recommendation on the limits for concentrations of radionuclides in the environment. On the | ||
Line 2,146: | Line 2,146: | ||
the 30th year of discharge) from external and internal exposure to the most exposed individuals in a hypothetical | the 30th year of discharge) from external and internal exposure to the most exposed individuals in a hypothetical | ||
critical group is to be performed. There are two steps (or iterations).<br> | critical group is to be performed. There are two steps (or iterations).<br> | ||
− | ''Step 1 of tier 1: Application of the ‘No dilution (no dispersion) model’''<br> | + | ''<big>Step 1 of tier 1: Application of the ‘No dilution (no dispersion) model’</big>''<br> |
The maximum predicted annual average radionuclide concentrations in Bq/m3 at the point of discharge in | The maximum predicted annual average radionuclide concentrations in Bq/m3 at the point of discharge in | ||
either air or water are to be used. Effective doses to a hypothetical critical group can be calculated by multiplying | either air or water are to be used. Effective doses to a hypothetical critical group can be calculated by multiplying | ||
Line 2,159: | Line 2,159: | ||
(see Section II.2.3) for a specific nuclear facility. If no compliance with the acceptance limit is found, the analysis | (see Section II.2.3) for a specific nuclear facility. If no compliance with the acceptance limit is found, the analysis | ||
should be refined with step 2.<br> | should be refined with step 2.<br> | ||
− | ''Step 2 of tier 1: Application of the ‘generic environmental model’''<br> | + | ''<big>Step 2 of tier 1: Application of the ‘generic environmental model’</big>''<br> |
The concentrations at the location nearest to the facility at which a member of the public will be likely to | The concentrations at the location nearest to the facility at which a member of the public will be likely to | ||
have access, or from which a member of the public may obtain food or water, are needed in order to see whether | have access, or from which a member of the public may obtain food or water, are needed in order to see whether | ||
Line 2,750: | Line 2,750: | ||
| | | | ||
|- | |- | ||
− | + | !Total | |
| | | | ||
| | | | ||
Line 2,759: | Line 2,759: | ||
|} | |} | ||
<small>* - Normalized emissions in liquid effluents (0.01 for Pb-210 and Th-230; 0.02 for Ra-226; 0.3 for U-234 and U-238) contribute negligibly to the collective dose.<br> ** - Annual activity released; the rate of activity is assumed to remain constant over more than 10 000 years.<br>*** - Dose commitment corresponding to a five year release.<br>**** - Dose commitment corresponding to a 10 000 year release.</small> | <small>* - Normalized emissions in liquid effluents (0.01 for Pb-210 and Th-230; 0.02 for Ra-226; 0.3 for U-234 and U-238) contribute negligibly to the collective dose.<br> ** - Annual activity released; the rate of activity is assumed to remain constant over more than 10 000 years.<br>*** - Dose commitment corresponding to a five year release.<br>**** - Dose commitment corresponding to a 10 000 year release.</small> | ||
+ | |||
+ | |||
+ | {| class="wikitable" | ||
+ | |+Table 16. Selected normalized radiological emissions from mixed oxide fuel fabrication | ||
+ | !Type of emission | ||
+ | !Value | ||
+ | |- | ||
+ | |Annual atmospheric emissions (Bq/a) | ||
+ | |10<sup>7</sup> - 10<sup>8</sup> | ||
+ | |- | ||
+ | |Normalized annual emissions (Bq/(GW(e)∙a)) | ||
+ | |10<sup>7</sup> - 10<sup>8</sup> | ||
+ | |} | ||
+ | |||
+ | {| class="wikitable" | ||
+ | |+Table 17. Doses from mixed oxide fuel fabrication [30] | ||
+ | !Effective dose | ||
+ | !Existing plants (experience) | ||
+ | !Modern plants (design) | ||
+ | |- | ||
+ | |Annual effective dose per monitored worker (Sv/a) (average) | ||
+ | |0.007 | ||
+ | |0.003 | ||
+ | |- | ||
+ | |Collective effective dose per produced mixed oxide tonne (man Sv/t) | ||
+ | |0.07 | ||
+ | |0.005-0.020 | ||
+ | |- | ||
+ | |Normalized collective effective dose (man Sv/(GW(e)∙a)) | ||
+ | |2.0 | ||
+ | |0.15 - 0.60 | ||
+ | |} | ||
+ | |||
+ | {| class="wikitable" | ||
+ | |+Table 18. Selected normalized radiological emissions and doses from fuel reprocessing (oxide fuel) | ||
+ | !Time period | ||
+ | !Monitored workers (thousands) | ||
+ | !Annual collective effective dose (man Sv) | ||
+ | !Annual effective dose per monitored worker (mSv) | ||
+ | |- | ||
+ | |1975 - 1979 | ||
+ | |0.1 | ||
+ | |0.36 | ||
+ | |4.0 | ||
+ | |- | ||
+ | |1980 - 1984 | ||
+ | |1.0 | ||
+ | |2.4 | ||
+ | |2.3 | ||
+ | |- | ||
+ | |1985 - 1989 | ||
+ | |4.0 | ||
+ | |5.7 | ||
+ | |1.4 | ||
+ | |} | ||
+ | Values of actual annual emissions from currently installed nuclear facilities can be found in several publications | ||
+ | (e.g. Ref. [98]). Annex C of Ref. [98] includes mining and milling (emissions per tonne of produced uranium, using | ||
+ | a model mine and mill), uranium enrichment, fuel fabrication, nuclear reactor operation, fuel reprocessing and | ||
+ | solid waste disposal. For power plants, power production and emission data in recent years are included for noble | ||
+ | gases, tritium, <sup>131</sup>I and particulates to air, and tritium and other radionuclides to water. Reference [98] also reports | ||
+ | the specific normalized collective effective doses in terms of the units man Sv/GW∙a. <br> | ||
+ | The normalized collective effective dose of 7.5 man Sv/GW∙a is calculated in Ref. [98] for mill tailings of | ||
+ | radon releases integrated over 10 000 years. Incidentally, the releases to groundwater from low and intermediate | ||
+ | disposal in shallow depositories of waste from reactor operation produce 0.5 man Sv/GW∙a, mainly from <sup>14</sup>C. <br> | ||
+ | A review of five year annual emissions from individual nuclear power plants in the European Union is reported | ||
+ | in Ref. [99]. Data were taken from plant annual reports and comprise, for airborne emissions: the noble gases, | ||
+ | tritium, <sup>131</sup>I and other beta and gamma emitters; for emissions to water: tritium, other beta and gamma emitters | ||
+ | (including spectra of individual radionuclides when available) and alpha emitters when available. Reference [99] | ||
+ | also reports discharges from reprocessing sites in Europe, La Hague and Marcoule (France), and Sellafield and | ||
+ | Dounreay (United Kingdom), which include aerial discharges of tritium, total beta and gamma emitters (without | ||
+ | tritium), <sup>14</sup>C, <sup>85</sup>Kr, iodine isotopes and total alpha emitters (individual radionuclides are also given for Dounreay | ||
+ | and Sellafield). Data on liquid discharges from reprocessing sites include tritium, total beta and gamma emitters | ||
+ | (without tritium), <sup>14</sup>C and total alpha emitters. Liquid discharge spectra are also included for the four sites. Portions | ||
+ | of discharge limits are indicated for individual species or groups of radionuclides. <br> | ||
+ | Emission data as well as doses to critical groups can be found in annual environmental reports of several | ||
+ | nuclear facilities, e.g. for reprocessing at La Hague, the annual report [55] includes radioactive, as well as | ||
+ | non-radioactive emissions, and the site authorization levels for all discharge classes. Airborne radioactive emissions | ||
+ | given in Ref. [55] are tritium, iodine, noble gases including <sup>14</sup>C, <sup>85</sup>Kr, other beta and gamma emitters, as well as | ||
+ | alpha emitters. <br> | ||
+ | Reference [100] provides data on radioactive emissions to the sea: tritium, iodine, <sup>134</sup>Cs, <sup>137</sup>Cs, <sup>14</sup>C, | ||
+ | <sup>60</sup>Co, <sup>106</sup>Ru and <sup>90</sup>Sr, other beta and gamma emitters, as well as alpha emitters. Non-radiological emissions to | ||
+ | air comprise: CO, NO<sub>X</sub>, SO<sub>2</sub> and particulates. Reference [101] reports volatile organic compounds, ammonia, | ||
+ | calcium, hydrogen chloride, hydrogen fluoride, silicon and sodium, and the heavy metals iron, nickel, titanium | ||
+ | and vanadium. Non-radiological emissions to water comprise: aluminium, ammonia, barium, cadmium, chemical | ||
+ | oxygen demand, chromium, cobalt, fluorine, hydrazine, iron, lead, manganese, mercury, nickel, nitrate, nitrite, | ||
+ | phosphorous, sulphur, tributylphosphate, zinc and zirconium. Annual amounts of industrial and hazardous wastes | ||
+ | are presented. In addition, the demands of chemicals (sodium hydroxide, nitric acid, formaldehyde), water, fossil | ||
+ | fuels and electricity are provided, which can be useful for life cycle accounting of secondary material flows [102]. <br> | ||
+ | The assessment of radiological impacts, in terms of biota dose rates and their related potential biological | ||
+ | effects on marine biota arising from radioactive discharges to the sea (as liquid effluents) of the La Hague facility | ||
+ | can provide a part of the necessary input data for the INPRO assessment of a planned NES facility [103, 104]. In | ||
+ | Ref. [103], a representative set of local marine biota is selected for assessment. The guidance values are based | ||
+ | on Refs [38, 41], and on a review of the database developed in the FASSET project [105]. The derived generic | ||
+ | guidance values are similar to those published in the MARINA II study on radioactive discharges into north | ||
+ | European marine waters, concentrations of radionuclides in the environment and assessment of their impact [106]. | ||
+ | The study provides dose rate results for selected marine biota categories for the coastal areas of La Hague and | ||
+ | Sellafield. MARINA II also includes generic guidance values for the protection of marine biota [104, 106]. The | ||
+ | estimated marine biota dose rates are low (by at least two to three orders of magnitude) compared with the lowest | ||
+ | generic guidance values for the protection of populations of marine biota [103].<br> | ||
+ | A summary of an environmental assessment of Bruce, a Canadian nuclear power plant, can be found in | ||
+ | Ref. [107], which also includes non-radioactive stressors that are, however, producing negligible impacts.<br> | ||
+ | A generic comparative study has been performed by the OECD Nuclear Energy Agency (NEA) on the | ||
+ | radiological impact of spent nuclear fuel options [108]. The options considered were a pressurized water reactor | ||
+ | (PWR) with an open (once through) uranium fuel cycle and the same PWR with monorecycling of uranium fuel. | ||
+ | Generic release rates of radionuclides of all fuel cycle facilities including the power plant can be found in Ref. [108]. | ||
+ | |||
+ | ====Comprehensive environmental studies==== | ||
+ | The nuclear fuel cycle has been the object of a few life cycle assessment (LCA) studies focused on greenhouse | ||
+ | gases or energy expenditures, but relatively few comprehensive studies have been published on current nuclear fuel | ||
+ | cycles, and even fewer on future NESs. Known published examples of comprehensive studies on current operating | ||
+ | NESs are given below.<br> | ||
+ | Within the Externalities of Energy (ExternE) project of the European Commission, the French nuclear fuel | ||
+ | cycle was evaluated. All stages were included in the assessment, from mining uranium ores through to waste | ||
+ | disposal. The general methodology adopted in the study was the ‘impact pathway analysis’, modelling the path | ||
+ | from discharges into the environment through dispersion and deposition to potential doses to humans. The damages | ||
+ | (collective doses to workers and the public) were converted to costs, using a single framework devised for all the | ||
+ | fuel cycles considered in the ExternE project [109–111].<br> | ||
+ | The Swiss LCA study on current (reference year 2000) European energy systems [112–114] included the | ||
+ | nuclear fuel cycles for PWRs, boiling water reactors (BWRs) and country specific LWR mixes [102, 113]. Both | ||
+ | open and closed fuel cycles (one time reprocessing of spent fuel, at equilibrium) were analysed. All stages of | ||
+ | the front and back ends were included. Approximately a thousand elementary environmental flows (requirements | ||
+ | as well as emissions) per unit of net electricity produced at the power plant are available in the ecoinvent LCA | ||
+ | database, described in Section II.6.2. Known examples of comprehensive environmental assessments of possible | ||
+ | future nuclear fuel cycles are given below.<br> | ||
+ | An extrapolation of LCA data of currently installed technology (Swiss LWR) to new designs (AP600 and | ||
+ | advanced BWRs) was performed in Ref. [115]. Also taken into account after a priority analysis were: improvements | ||
+ | in mill tailing management; centrifuge/atomic vapour laser isotope separation enrichment plants substituting | ||
+ | the units based on the gaseous diffusion process once they were phased out; and reduction of waste volumes at | ||
+ | reprocessing. A semistatic approach was defined to calculate cumulative burdens at discrete time points. | ||
+ | An assessment of five different scenarios (once through, single plutonium recycling, plutonium | ||
+ | multirecycling, 45% thermal 55% breeder, and breeder burning neptunium, americium and copper homogeneously | ||
+ | mixed with plutonium) within the twenty-first century in the French context was published in 2004 [116]. The EPR | ||
+ | and European fast reactor types were modelled. Conventional emissions and the health effects of ionizing radiation | ||
+ | (local impacts) were analysed. Application of LCA, covering the global environmental analysis part of the study, | ||
+ | actually focused on a few emission species to air, was complemented by the assessment of local and regional | ||
+ | radiological impacts on humans in terms of annual doses, and by the inventorying of nuclear materials using the | ||
+ | material flow accounting tool COSI.<br> | ||
+ | A project of the European Commission addressed future technologies up to the year 2050, integrating LCA | ||
+ | with external cost estimation and an optimization energy economy code (Times-MARKAL). The nuclear industry is | ||
+ | represented with Generation III and Generation IV power plants, in addition to fossil fuel and renewable technology | ||
+ | power plants. A multicriteria decision assessment (MCDA) methodology will be used, as well as external costings | ||
+ | for comparison and ranking of the technology options. | ||
+ | |||
+ | ====Life cycle assessment ecoinvent database==== | ||
+ | The commercial, centralized, web based LCA database ecoinvent was developed and implemented by the | ||
+ | Swiss Centre for Life Cycle Inventories and supported by Swiss Federal Offices. It has been available on-line since | ||
+ | September 2003.<br> | ||
+ | The aim of developing the centralized LCA ecoinvent database was to achieve consistency throughout | ||
+ | different life cycle inventory (LCI) databases maintained by the participating organizations. The sectors included | ||
+ | are: energy systems (Paul Scherrer Institute); materials and metals, waste treatment and disposal (Swiss Federal | ||
+ | Laboratories for Materials Testing and Research); transport systems and chemicals (Swiss Federal Institute of | ||
+ | Technology in Zurich); and agricultural products (Swiss Federal Research Station for Agroecology and Agriculture). | ||
+ | About 2750 individual processes (datasets) have been modelled and about 1000 elementary environmental flows | ||
+ | inventoried, including emissions to air, water, soil, solid wastes, land use, and biotic and abiotic resources. The | ||
+ | environmental cumulative burdens associated with the processes are integrated based on an algorithm, thus | ||
+ | reflecting the interactions of industrial activities within an economy system. In addition to LCI, ecoinvent also | ||
+ | includes the results of LCA, using current methods developed by different organizations [117].<br> | ||
+ | The assessed energy systems, constituting about half of the processes available in the database, include | ||
+ | electricity and heating systems [112, 113]. Electricity transmission and distribution, as well as country specific | ||
+ | production and supply mixes, have also been modelled. Fossil fuel, nuclear and renewable energy systems | ||
+ | associated with Swiss and European power plants, boilers and cogeneration plants have been assessed, reflecting | ||
+ | conditions around the reference year 2000.<br> | ||
+ | The nuclear fuel cycles modelled in ecoinvent are those associated with power generation at LWRs currently | ||
+ | installed in the Union for the Co-ordination of Transmission of Electricity (UCTE) [102, 114]. The focus was on | ||
+ | two 1000 MW units operating in Switzerland: Gösgen PWR and Leibstadt BWR. The conditions of the fuel cycles | ||
+ | for the Swiss reactors were assessed, modelled and extrapolated to France, Germany and the UCTE (which can | ||
+ | be assumed to be representative of LWRs in western Europe). In addition to describing the nuclear cycle as such, | ||
+ | the assessment served the estimation of cumulative environmental burdens of European electricity mixes, along | ||
+ | with other electricity systems in ecoinvent. The stages separately modelled were: mining, milling, conversion, | ||
+ | enrichment (diffusion and centrifuge), fuel fabrication, PWRs, BWRs, national mixes of PWRs and BWRs, | ||
+ | reprocessing, spent fuel conditioning, interim storage, low level radioactive waste depository and geological final | ||
+ | repositories. | ||
+ | |||
+ | ==Appendix III== | ||
+ | <big>'''BASIC CONCEPTS FOR OPTIMIZING MANAGEMENT OPTIONS FOR REDUCTION OF ENVIRONMENTAL IMPACT'''</big><br> | ||
+ | ===As low as reasonably practicable=== | ||
+ | ====Concept==== | ||
+ | The concept of ALARP (economic and social factors taken into account) is used to define an AL for several | ||
+ | INs in different areas of the INPRO methodology, especially for the environment and waste management. | ||
+ | [[File:FIG. 5. The ALARP concept (21)..png|thumb|FIG. 5. The ALARP concept [21].]] | ||
+ | The concept is illustrated in Fig. 5. As shown, the risk (symbolized by the triangle) is divided into three | ||
+ | regions: a broadly acceptable region, a tolerable region where a process for ALARP has to be used and an | ||
+ | unacceptable region.<br> | ||
+ | As a first step of the ALARP concept to be applied within the INPRO methodology, the boundary values of | ||
+ | these three regions have to be determined: the boundary between the tolerable and the unacceptable regions, usually | ||
+ | called a basic limit (BL); and the boundary between the tolerable and broadly acceptable regions, sometimes called | ||
+ | a basic objective (BO). The next step is to confirm that the value of the indicator of an NES is within the ALARP | ||
+ | region that is below the BL. The last step is to confirm that in an optimization analysis, all measures to reduce | ||
+ | the specific risk have been taken into account by the designer up to a level where the costs for these measures | ||
+ | would have become ‘grossly disproportional’ to the benefit gained. It is important to note that, in the case when | ||
+ | the indicator of an NES has a value in the broadly acceptable region below the boundary BO, no further effort is | ||
+ | necessary to fulfil the ALARP concept.<br> | ||
+ | BL and BO values may be specified for specific indicators in national regulations or as an outcome of an | ||
+ | EIA, or it may be necessary to infer such values from other evidence such as: licence conditions; actions planned, | ||
+ | under way or completed to remediate an existing situation or improve a given practice; presentations at national | ||
+ | or international conferences; publications in peer reviewed journals, the IAEA Safety Standards Series and other | ||
+ | IAEA publications; and the work of other organizations such as the ICRP, the NEA and the European Commission. | ||
+ | When a BL or a BO is deduced from such evidence, the rationale for doing so needs to be clearly stated to ensure | ||
+ | transparency. While national and international consensus may not exist and may be slow to emerge, suggesting a | ||
+ | value for a BO and the associated rationale would be expected to make a valuable contribution to the process of | ||
+ | national and international consensus building. | ||
+ | |||
+ | ====Application of the ALARP concept by a technology developer==== | ||
+ | The flow chart in Fig. 6 exemplifies the procedure that a technology developer (designer) could follow | ||
+ | to confirm compliance of an NES under development with the INPRO methodology CR3.1 in the area of | ||
+ | environmental stressors (optimization of the measures to reduce environmental impact).<br> | ||
+ | The quantity, optimized in an ALARP analysis, could be an individual stressor level, a weighted combination | ||
+ | of stressor levels (e.g. estimated dose) or some other risk related function of the system parameters. The values of | ||
+ | BL and BO are to be set on the basis of expert judgement, best technology available, regulatory clearance levels or | ||
+ | other information.<br> | ||
+ | The depth that the ALARP analysis can reach depends on the maturity level of the NES assessed. For early | ||
+ | designs that are still at the conceptual stage, the optimization process could be guided by establishing a figure of | ||
+ | merit for acceptable limits and restrict the ALARP procedure to stressors (radioactive as well as non-radioactive, | ||
+ | if relevant) that have been identified as being the most significant or of the highest priority. Further reductions | ||
+ | of the number of stressors could be accepted only if they have been appropriately justified by the designer, with | ||
+ | evidence provided in the analysis report.<br> | ||
+ | The ALARP concept might be applied to the whole NES under development, for which adequate BL/BO | ||
+ | values would need to be defined. In the case when the optimization occurs both at the individual facility (component) | ||
+ | level and at the whole system level, care needs to be taken to ensure that double counting does not occur. As an | ||
+ | example, in addition to a BL and a BO on <sup>14</sup>C emitted from a reactor or a reprocessing facility, a BL and BO for the | ||
+ | aggregated <sup>14</sup>C from the entire NES under development, including reprocessing and waste treatment facilities, can | ||
+ | also be set (see also the discussion below on the reduction of collective doses).<br> | ||
+ | In the following, the proposed approach for each facility (component) is described in more detail, as it | ||
+ | corresponds to the way that ALARP is mostly applied in current practices.<br> | ||
+ | If the analysis is performed in several stages for addressing successive levels of maturity of the NES being | ||
+ | developed or for considering possible changes in the regulations of the Member State or in international standards, | ||
+ | the BL and BO values might also need to be redefined, and the ALARP analysis may become more refined and | ||
+ | complex.<br> | ||
+ | If the NES being developed is just at its conceptual stage, the ALARP demonstration may be reduced to a | ||
+ | discussion or a series of statements (illustrations) aimed at showing that modifications to the system that could | ||
+ | reduce these stressors have been considered, and that these stressors cannot practicably be reduced further. At the | ||
+ | very least, the developer (designer) should acquire evidence that sufficient thought has gone into evaluating the | ||
+ | potential of adjustments in the NES facilities and processes to reduce environmental effects. The descriptive | ||
+ | demonstration, adequately reported in the analysis report, should be finalized in the following phases of design | ||
+ | development. Reference [72] explains that the requirement for risks to be ALARP “is a requirement to take all | ||
+ | measures to reduce risk where doing so is reasonable. In most cases this is not done through an explicit comparison | ||
+ | of costs and benefits, but rather by applying established relevant good practice and standards.”<br> | ||
+ | Hence, it is expected that if an analysis of more refined stages of the design process is performed, this should | ||
+ | verify ALARP for the same component(s)/stressor(s), and, if the design is changed, track the reasons for technical | ||
+ | modifications in relation to the previous ALARP analysis. | ||
+ | [[File:FIG. 6. Proposed method for ALARP applied in sequence to all facilities (components) of a nuclear energy system (NES) or,.png|thumb|FIG. 6. Proposed method for ALARP applied in sequence to all facilities (components) of a nuclear energy system (NES) or, if necessary, to groups of facilities or/and to the whole NES [1]. ALARP — as low as reasonably practicable; BL — basic limit; BO — basic objective; RD&D — research, development and demonstration.]] | ||
+ | If a facility, or the whole NES, is at a more advanced state of development, ALARP may require the application | ||
+ | of tools for aiding in decisions such as CBA or MCDA [72, 118]. It may happen that ALARP implementation in a | ||
+ | given facility may be challenging (stressors must be below BL for licensing) because of compensating effects across | ||
+ | the spectrum of stressors of the facility itself or group of facilities of the NES. Reference [72] notes that “where | ||
+ | there are several risks which interact, whether arising from a single hazard or from different connected hazards, | ||
+ | there may be a need for balancing to achieve the best overall solution.” In this case, the developer (designer) should | ||
+ | demonstrate and document that ALARP is fulfilled at a higher level by analysing groups of components or the | ||
+ | NES as a whole (see Fig. 6). A sensitivity analysis may be useful to show the robustness of the adopted technical | ||
+ | solution by varying key parameters (see the discussion below on the proportion factor between costs and risks). | ||
+ | CBA and/or MCDA need to be applied considering all aspects within the boundary of the assessment.<br> | ||
+ | With reference to Fig. 6, what ‘gross disproportion between costs and risk reduction’ means for application, | ||
+ | no algorithm has been defined by the risk and safety authorities adopting ALARP, rather only a general guidance | ||
+ | for judgement to allow flexibility on a case by case basis. For current technology, ALARP involves consideration | ||
+ | of good practices [52]. However, this may change owing to innovation, which is improving the degree of control, | ||
+ | cost changes or knowledge about hazards [72]. For new technologies in an NES, evolving good practices could be | ||
+ | followed to start with, and then consideration given to whether there are alternative processes or procedures that | ||
+ | could be performed to reduce the risk on the basis of first principles to compare the risk with the sacrifice involved | ||
+ | in further reducing it. In many instances, such ‘first principles’ comparisons can be carried out qualitatively, | ||
+ | i.e. using common sense or exercising professional judgement or experience [52]. In the case when the costs are | ||
+ | definitively very high and the reduction in risk is just marginal, then ALARP is likely demonstrated, and no further | ||
+ | improvements need to be designed or implemented. Conversely, in most cases where the improvements identified | ||
+ | are simple or cheap to implement and the risk significantly reduced, these improvements are required, and ALARP | ||
+ | can be considered to be achieved without further analysis [52].<br> | ||
+ | For less clear cut situations, which are most likely for innovative technologies, a more formal CBA may | ||
+ | provide additional insight to help to arrive at a judgement. In a CBA, both risk and sacrifice are converted into | ||
+ | monetary terms. In a standard CBA, the rule applies that the measure should be adopted if benefits outweigh costs. | ||
+ | However, in ALARP judgements, the measure has to be adopted unless the sacrifice is grossly disproportionate | ||
+ | to the risk. Therefore, “the costs can outweigh benefits and the measure could still be reasonably practicable to | ||
+ | introduce. How much costs can outweigh benefits before being judged grossly disproportionate depends on factors | ||
+ | such as how big the risk is to begin with (the larger the risk, the greater can be the disproportion between the cost | ||
+ | and risk)” [52].<br> | ||
+ | Although individual doses to members of the critical groups are used for establishment of standards, hence | ||
+ | of BL and BO values, for the CBA, the total social costs of all emissions (radiological as well as non-radiological) | ||
+ | have to be taken into account. Therefore, it is the collective dose (see Appendix IV) that should be used. The scope | ||
+ | of the analysis depends on the choice by the developer (designer) of the consistency within the analysis. Ideally, | ||
+ | externalities should be evaluated considering the LCA of the entire cycle, thus including secondary burdens from | ||
+ | flows of material and energy into the system. However, in the case when the options to be addressed in the ALARP | ||
+ | procedure would not meaningfully change the secondary contributions in relative terms, the analysis can be | ||
+ | restricted to the effect of primary (or direct) burdens only. Studies of external costs for current nuclear technologies | ||
+ | can be found in Refs [110, 115, 119, 120].<br> | ||
+ | The ALARP process should also take into account risks that may affect future generations, as they may stem | ||
+ | from radioactive waste management and decommissioning. Reference [72] notes that “risks should be assessed in a | ||
+ | holistic manner and not restricted to part of the overall time period or part of a process.” Further elaboration points | ||
+ | out that: “Although it could be argued that the next few generations may gain some indirect benefit, the uncertainty | ||
+ | of how they will view the risks left to them (and indeed the uncertainty of any benefits further into the future) | ||
+ | argues for a precautionary approach and hence a particularly stringent demonstration that risks are indeed ALARP.” | ||
+ | Considering that the demonstration of long term sustainability for an NES is the ultimate objective of an INPRO | ||
+ | assessment, the requirement of consideration of effects into the future should be even stronger when applied to an | ||
+ | NES under development.<br> | ||
+ | The following information, although referring to the ALARA approach, may also be of interest for ALARP. | ||
+ | The DOE recommends that for the purpose of comparing ALARA alternatives for operational systems, the lifetime | ||
+ | of the facility generally is a basis for truncating collective dose estimates, temporally [118]. However, where | ||
+ | cleanup, restoration and waste management activities are necessary, the time frame for the analysis can be much | ||
+ | longer. In particular, for long lived radionuclides, integration times may be determined by the uncertainties in | ||
+ | scenarios and by the physical parameters affecting dose rates. The DOE states that, typically, it is only appropriate | ||
+ | to conduct quantitative comparisons for a few hundred years or less. Although evaluating doses for periods of | ||
+ | up to a thousand years may provide useful information, periods beyond this length of time should not be used in | ||
+ | quantitative ALARA assessments [118]. However, although this position may be adopted for an analysis, provided | ||
+ | it is justified by the nature and amounts of the releases, consideration of longer integration times may be useful in | ||
+ | other parts of the analysis.<br> | ||
+ | Reference [72] defines that: “The safety benefit of the improvement is the incremental difference in risk | ||
+ | between the two estimates in terms of the detriments (i.e. all the adverse consequences) and their likelihoods, | ||
+ | summed over the remaining life of the facility.” This includes detriments from normal operation such as radiation | ||
+ | exposures of workers and the public; detriments from accidents which again includes radiation exposures to | ||
+ | the public and workers but also includes other detriments such as the need to decontaminate areas, evacuation, | ||
+ | relocation and food restrictions. Valuation of detriments is a subject of much discussion and research [72]. | ||
+ | The UK HSE [71] uses £1.5 million (2009) for the value of preventing a statistical fatality. In the case of | ||
+ | death caused by cancer, the HSE takes the view that people are prepared to pay a premium. Thus, a higher value, | ||
+ | such as twice that defined above, should be used. It is suggested that this higher value is also appropriate for all | ||
+ | radiation deaths.<br> | ||
+ | In the European study ExternE [109, 120], the value for average social (or external) costs in Europe attributed | ||
+ | to any cancer, whatever its origin and outcome (fatal or non-fatal), is €2 million. In the case where an assessment is | ||
+ | performed for a different country, if ‘willingness to pay’ (WTP) estimates are lacking, values established in Europe | ||
+ | or the United States of America can be adjusted to specific conditions using the ratio of the purchasing power | ||
+ | parity gross national product per capita. The adjustments are based on the assumption that there is an ‘elasticity’ of | ||
+ | WTP with respect to real income. An example of the application of this approach to the assessment of electricity | ||
+ | scenarios (including nuclear) for the Shandong Province, in China, can be found in Ref. [121]. | ||
+ | Use of external cost calculations implies verification, to the latest available information on damage | ||
+ | functions and external costs in publications, and detailed reporting on the assumptions/options used or possible | ||
+ | modifications/adaptations.<br> | ||
+ | The following applies to ALARA as implemented in the United States of America. However, the information | ||
+ | may also be useful for ALARP assessments. For the costs associated with units of doses, the linear relationship of | ||
+ | health effects with dose is postulated, thus making the monetary value independent of the magnitude of individual | ||
+ | doses. Several rationales have been adopted for the estimation of the monetary value of a unit of collective dose or | ||
+ | other effects of non-radioactive substances, which can be found in the literature. The use of WTP, i.e. the willingness | ||
+ | to commit resources to avoid the negative impact, is considered here. As an example of application, in 1995, the | ||
+ | NRC [122] re-evaluated the monetary equivalent value and issued regulatory analysis guidelines that recommend | ||
+ | US $2000/man rem (US $200 000/Sv) as the current monetary equivalent value for converting collective dose | ||
+ | to US dollars [118]. The DOE accepted the NRC recommendation, and, because of the uncertainty in the values | ||
+ | used in ALARA analyses, further elaborated that detailed ALARA evaluations use the range for comparing | ||
+ | alternatives. The DOE evaluations to support ALARA analyses apply monetary equivalents for a man rem in the | ||
+ | range US $1000–6000 with a nominal value of US $2000 [118, 123]. Assuming that one man rem corresponds to | ||
+ | a potential risk of 5 × 10<sup>−4</sup> radiation induced fatal health effects (generally cancers) for the population, the range | ||
+ | recommended by the DOE would correspond to a range of US $2 million to US $12 million per hypothetical | ||
+ | radiation induced cancer death averted [118].<br> | ||
+ | In cases where, in addition to health (and environmental) risks and costs of measures to reduce them, several | ||
+ | other non-health detriments are identified, these should also be included in the analysis with societal costs when | ||
+ | appropriate and feasible. These effects can be physical (e.g. waste heat discharge, noise or damage from potential, | ||
+ | very low frequency/high consequence accidents — for the latter, see Ref. [120]) or sociopolitical (e.g. aversion, | ||
+ | opposition or proliferation). Some may be quantified in monetary terms and could be included in the CBA. | ||
+ | However, in most cases, such quantification has strong subjective elements, i.e. weighting factors may intervene | ||
+ | (see discussion in Ref. [118], p. 18). Therefore, in cases where non-monetary aspects are identified or anticipated as | ||
+ | a crucial part of the decision on which measures identified in an ALARP process should be implemented, a formal | ||
+ | MCDA may be best applied.<br> | ||
+ | As mentioned in Ref. [72], if the risks (given by frequency multiplied by the consequences) are high, then the | ||
+ | demonstration of ALARP should be more rigorous than if the risk is low. Where risks are high, the consequences | ||
+ | should be weighted more than the frequency, and the ALARP demonstration should be accurate. | ||
+ | The technical assessment guide of the Office for Nuclear Regulation [72] provides specific guidance for | ||
+ | inspectors of nuclear installations on what they should expect of a licensee in meeting legal requirements to reduce | ||
+ | risks to ALARP. It suggests, on the basis of previous experience, a disproportion factor between costs and risk | ||
+ | reduction benefits of up to three for workers, whereas for risks to the public, the factor would depend on the level | ||
+ | of risk, suggesting a factor of about two for low risks and about ten for higher risks. These factors may be applied | ||
+ | in an analysis, unless other rationale are provided.<br> | ||
+ | Owing to the uncertainties at various levels in the system under scrutiny and in the methods used for the | ||
+ | prediction of releases and associated consequences, it is suggested in Ref. [72] to base the judgement on the | ||
+ | appropriateness of the proportion factor on the robustness of the analysis. This factor should also be one of the | ||
+ | parameters to be varied in a sensitivity analysis<br>. | ||
+ | Considering that, usually, the scope of an environmental analysis includes also anticipated operational | ||
+ | occurrences, it is useful that the National Radiological Protection Board (NRPB) has produced guidance relating | ||
+ | to the dose saved resulting from routine exposure or minor incidents, i.e. stochastic effects, up to about 100 mSv. | ||
+ | The NRPB recommends that an increasing multiplier is used in ALARP demonstrations as the dose increases, | ||
+ | and also recommends the HSE Nuclear Safety Directorate to assess licensees’ submissions using this approach. | ||
+ | Reference [72] suggests that a factor (for costs outweighing risks) of less than ten in the vicinity of the intolerable | ||
+ | region is unlikely to be acceptable and, for hazards that can cause large consequences, the factor may need to be | ||
+ | larger still. This requirement may be understood as being even more pronounced for an NES under development, | ||
+ | especially at the early design stages for which the uncertainties may be predominant on the cost differences | ||
+ | calculated for risk reduction measures. The recommendation of Ref. [72], discussed above, to include the proportion | ||
+ | factor within the parameters to be varied in a sensitivity analysis is repeated here. | ||
+ | |||
+ | ====Example of application to a reprocessing facility==== | ||
+ | One of the rather few examples of the application of ALARP to a facility of a current NES [84] reports on the | ||
+ | Environment Agency’s re-examination of the Sellafield reprocessing plant’s radioactive discharge authorizations | ||
+ | from 2000 to 2002. This example would have the potential to also serve for assessing the ALARP concept of future | ||
+ | reprocessing plants. However, the information available in Ref. [84] is rather limited, and is therefore insufficient | ||
+ | for full understanding of the procedure used (e.g. missing information on risk levels before implementation of | ||
+ | ALARP, how the optimized actions were justified and on the costs involved).<br> | ||
+ | Table 19 presents the doses to the critical groups caused by radioactive discharges from the Sellafield | ||
+ | reprocessing plant in 2001 [84]. | ||
+ | {| class="wikitable" | ||
+ | |+Table 19. Critical group doses from 2001 discharges from the sellafield site [84] | ||
+ | !colspan="2"|Aerial effluent discharges | ||
+ | !colspan="2"|Liquid effluent discharges | ||
+ | |- | ||
+ | !Radionuiclide | ||
+ | !Critical group dose (adult) (μSv) | ||
+ | !Radionuclide | ||
+ | !Critical group dose (seafood consumer) (μSv) | ||
+ | |- | ||
+ | |Ar-41 | ||
+ | |31 | ||
+ | |Tc-99 | ||
+ | |23 | ||
+ | |- | ||
+ | |Sr-90 | ||
+ | |11 | ||
+ | |C-14 | ||
+ | |5 | ||
+ | |- | ||
+ | |Ru-106 | ||
+ | |3 | ||
+ | |Cs-137 | ||
+ | |4 | ||
+ | |- | ||
+ | |Cs-137 | ||
+ | |3 | ||
+ | |Ru-106 | ||
+ | |2 | ||
+ | |- | ||
+ | |Pu alpha | ||
+ | |2 | ||
+ | |Sr-90 | ||
+ | |1 | ||
+ | |- | ||
+ | |C-14 | ||
+ | |1 | ||
+ | |Co-60 | ||
+ | |1 | ||
+ | |- | ||
+ | |Kr-85 | ||
+ | |1 | ||
+ | |Others | ||
+ | |1 | ||
+ | |- | ||
+ | |I-129 | ||
+ | |1 | ||
+ | | | ||
+ | | | ||
+ | |- | ||
+ | |Am-241 | ||
+ | |1 | ||
+ | |Am-241* | ||
+ | |50 | ||
+ | |- | ||
+ | |I-131 | ||
+ | |1 | ||
+ | |Pu alpha* | ||
+ | |28 | ||
+ | |- | ||
+ | |Others | ||
+ | |3 | ||
+ | |Pu-241* | ||
+ | |4 | ||
+ | |- | ||
+ | !Total | ||
+ | |58 | ||
+ | !Total | ||
+ | |119 | ||
+ | |} | ||
+ | <small>* - Historic contribution from discharges prior to the Site IonExchange Effluent Plant (SIXEP) and the Enhanced Actinide Removal Plant (EARP) have been introduced.</small> | ||
+ | The strategy of the operator, British Nuclear Fuels (BNFL), for controlling radioactive discharges from the | ||
+ | Sellafield site consists of:<br> | ||
+ | (a) Designing plants to minimize radioactive arisings, and subsequent discharges and disposals, where practicable | ||
+ | by: | ||
+ | *Employing processes that avoid the production of gaseous and liquid by-products, and that avoid solid waste generation (it is a general rule that because environmental impacts are greatest per unit activity for aerial releases, and are smallest for solid wastes, the design of the process reflects this hierarchy for the avoidance of generation of by-products). | ||
+ | *Proper design of abatement systems. | ||
+ | *Maximizing dispersion. | ||
+ | (b) Commissioning, operating and decommissioning plants, minimizing liquid and aerial discharges by means | ||
+ | of: | ||
+ | *Prioritization in terms of dose impacts on the public. “To ensure resources are used in the most cost-effective manner, BNFL concentrates its ongoing research and development work on reducing those discharges which result in the highest doses or highest chemical toxicity, focusing effort on those areas in which there is the greatest potential for minimising environmental impact. For radioactive substances, it is generally the policy to use critical group dose as the key determining factor, though consideration is also given to collective doses” [84]. Table 19 shows that as of 2001 discharges, only a few radionuclides make almost all doses to the critical groups; this helps to identify where to intervene first in order to reduce risks to the population. Incidentally, the total critical group doses are within the BO–BL range of 0.02–1 mSv, as requested by the HSE. | ||
+ | *Increasing the magnitude of operations by decreasing the magnitude of wastes generated for eventual disposal. | ||
+ | *Addressing specific airborne emitted species: decreasing discharges of <sup>41</sup>Ar, <sup>14</sup>C and <sup>129</sup>I. | ||
+ | *Addressing specific water-borne emitted species: abating <sup>137</sup>Cs, <sup>60</sup>Co, <sup>239</sup>Pu and <sup>99</sup>Tc. | ||
+ | (c) Developing new technologies.<br> | ||
+ | (d) Implementing appropriate management systems. | ||
+ | |||
+ | ===Best available techiques=== | ||
+ | ====Concepts==== | ||
+ | According to the Convention for the Protection of the Marine Environment of the North-East Atlantic [13]: | ||
+ | “The term ‘best available techniques’ means the latest stage of development (state of the art) of processes, | ||
+ | of facilities or of methods of operation which indicate the practical suitability of a particular measure for | ||
+ | limiting discharges, emissions and waste. In determining whether a set of processes, facilities and methods of | ||
+ | operation constitute the best available techniques in general or individual cases, special consideration shall be | ||
+ | given to:<blockquote> | ||
+ | (a) comparable processes, facilities or methods of operation which have recently been successfully tried out;<br> | ||
+ | (b) technological advances and changes in scientific knowledge and understanding;<br> | ||
+ | (c) the economic feasibility of such techniques;<br> | ||
+ | (d) time limits for installation in both new and existing plants;<br> | ||
+ | (e) the nature and volume of the discharges and emissions concerned.” | ||
+ | </blockquote> | ||
+ | Related to the application of BAT is the application of BEP, which is defined in the Convention [13] as | ||
+ | “the application of the most appropriate combination of environmental control measures and strategies”. | ||
+ | The Convention further states that [13]: | ||
+ | <blockquote> | ||
+ | “In determining what combination of measures constitute best environmental practice, in general or individual | ||
+ | cases, particular consideration should be given to:<br> | ||
+ | (a) the environmental hazard of the product and its production, use and ultimate disposal;<br> | ||
+ | (b) the substitution by less polluting activities or substances;<br> | ||
+ | (c) the scale of use;<br> | ||
+ | (d) the potential environmental benefit or penalty of substitute materials or activities;<br> | ||
+ | (e) advances and changes in scientific knowledge and understanding;<br> | ||
+ | (f) time limits for implementation;<br> | ||
+ | (g) social and economic implications.” | ||
+ | </blockquote> | ||
+ | In addition, BEP for a particular source will change with time “in the light of technological advances, | ||
+ | economic and social factors, as well as changes in scientific knowledge and understanding” [13]. Finally [13]: | ||
+ | <blockquote>“If the reduction of inputs resulting from the use of best environmental practice does not lead to environmentally acceptable results, additional measures have to be applied and best environmental practice redefined.”</blockquote> | ||
+ | Regardless of the approach that is used for determining the optimal option, it should be recognized that | ||
+ | judgements are required about the relative significance of the factors involved, which includes dialogue between | ||
+ | the regulatory body and the operator, as well as other interested parties, particularly those who might be affected by | ||
+ | the situation, facility or planned activity (e.g. local community representatives).<br> | ||
+ | Formal decision aiding techniques may be used in the optimization process, especially for nuclear fuel cycle | ||
+ | facilities. However, when the doses to the representative person are assessed to be very low (e.g. of the order of | ||
+ | 10 μSv/a or less), a formal analysis of the optimization of protection is generally not necessary. The magnitude | ||
+ | of exposure, the number of exposed individuals and the likelihood of exposure should be assessed for each of the | ||
+ | options being considered. For complex facilities, elements of the radiological assessments should be made available | ||
+ | at an early stage, and the development of the assessment may be an iterative process involving discussions between | ||
+ | the operator, the regulatory body and other interested parties. The assessment should be refined as the development | ||
+ | of the facility moves towards the stage at which an application for a licence for the planned facility is to be made | ||
+ | (e.g. a licence to operate). | ||
+ | |||
+ | ====Application of best available techniques in environmental impact assessment==== | ||
+ | BAT assessment is typically undertaken when making decisions regarding the development and | ||
+ | implementation of appropriate discharge standards to ensure environmental protection. This can include situations | ||
+ | when standards for the discharge of radioactive material are being developed, for planning the management and | ||
+ | disposal of radioactive waste, when authorizations are undergoing a significant amendment or when facilities are in | ||
+ | a sensitive environment.<br> | ||
+ | An example is provided here for the Gunnar Mine Site, which is an abandoned uranium mine and mill site | ||
+ | in northern Saskatchewan, Canada. The site was operated between the late 1950s and early 1960s under a less | ||
+ | stringent regulatory regime than today. Uranium ore was initially mined from an open pit from 1955 to 1961, and | ||
+ | later, from an underground operation between 1957 and 1963. The Gunnar Mine Site officially closed in 1964, with | ||
+ | little or no remediation of its facilities.<br> | ||
+ | A stepwise decision tree approach was developed and applied to identify all potentially feasible remedial | ||
+ | options, as part of the EIA process, and to map out key decision points during the licensing phase of the project, | ||
+ | when final remedial options were selected from the list of options. Although the example provides an illustration of | ||
+ | the approach for the remediation of the Gunnar Mine Site, the same approach can be used for optimization of the | ||
+ | options intended for environmental protection.<br> | ||
+ | A number of end points (or desired outcomes of the remediation efforts) were identified for the site, as | ||
+ | follows: | ||
+ | *The site does not pose unreasonable public health or environmental risks. | ||
+ | *Flora and fauna adjacent to the site are not significantly affected by contaminants. | ||
+ | *Traditional use of resources adjacent to the site can be safely conducted. | ||
+ | *The desire is to have the site managed through an institutional controls programme for long term care and maintenance. | ||
+ | |||
+ | =====''Screening framework for evaluation of remedial options''===== | ||
+ | In conducting the alternatives assessment, all available options were identified and evaluated, including all | ||
+ | aspects of each mine waste disposal alternative throughout the project life cycle, and all aspects of the project | ||
+ | (direct or indirect) that may contribute to the predicted impacts associated with each potential option [124, 125]. | ||
+ | The assessment considered the predicted quality and quantity of releases to the environment, and the corresponding | ||
+ | predicted effects on the environment. The assessment of alternatives also considered the full cost of each remedial | ||
+ | option throughout the project life cycle and its associated benefits, as an independent step from the technical review | ||
+ | of options.<br> | ||
+ | Assessment of the predicted quality and quantity of releases to the environment, and corresponding effects to | ||
+ | the environment, are addressed through characterization of contaminant concentrations and key contaminant flow | ||
+ | pathways, and subsequently inputting data into the human health and ecological risk assessment (HHERA).<br> | ||
+ | Evaluation of remedial options was conducted using a multitiered assessment approach based on | ||
+ | environmental and technical CR, utilizing the framework for assessment of alternatives proposed in Ref. [124], and | ||
+ | which is presented in Table 20. | ||
+ | {| class="wikitable" | ||
+ | |+Table 20. | ||
+ | !Step | ||
+ | !Definition | ||
+ | !Criteria | ||
+ | !Analysis | ||
+ | !Deliverable | ||
+ | |- | ||
+ | |1 | ||
+ | |Identify candidate alternatives | ||
+ | |Threshold criteria | ||
+ | |Mine waste disposal technologies | ||
+ | Mine waste disposal storage options | ||
+ | Mine waste disposal sites | ||
+ | |Summary table | ||
+ | Location maps | ||
+ | |- | ||
+ | |2 | ||
+ | |Prescreening assessment | ||
+ | |Prescreening criteria | ||
+ | |Prescreening evaluation | ||
+ | |Summary table | ||
+ | |- | ||
+ | |3 | ||
+ | |Alternative characterization | ||
+ | |Characterization criteria (accounts) | ||
+ | |Environmental criteria | ||
+ | Technical criteria | ||
+ | Project economic criteria | ||
+ | Socioeconomic criteria | ||
+ | |Summary tables | ||
+ | |- | ||
+ | |4 | ||
+ | |Multiple accounts ledger | ||
+ | |Evaluation criteria (subaccounts) | ||
+ | Measurement criteria (indicators) | ||
+ | |Qualitative value scales | ||
+ | |Summary tables | ||
+ | |- | ||
+ | |5 | ||
+ | |Value based decision process | ||
+ | |Scoring | ||
+ | Weighting | ||
+ | |Quantitative analysis | ||
+ | |Summary tables | ||
+ | |- | ||
+ | |6 | ||
+ | |Sensitivity analysis | ||
+ | |Reconsider weighting | ||
+ | |Quantitative analyses | ||
+ | |Summary tables | ||
+ | |- | ||
+ | |7 | ||
+ | |Document results | ||
+ | | | ||
+ | | | ||
+ | |Final report | ||
+ | |} | ||
+ | |||
+ | The summary of the major steps of the assessment provided in Ref. [125] specifies that these steps include: | ||
+ | *Development of a list of all possible candidate remedial options, with the input from stakeholders and without prior judgements; | ||
+ | *Screening of options to reduce the number and to provide assurance that any of the remaining options could prove to be the preferred option; | ||
+ | *Characterization of the remaining alternatives to ensure proper consideration of every aspect and nuance and the comparison of alternatives in a clear and concise format, to ensure complete transparency of the decision process; | ||
+ | *Identification of evaluation criteria that are linked to an effect and that easily differentiate the alternatives; | ||
+ | *Inclusion of quantitative value judgement in the decision process by scoring and weighting all evaluation criteria under the environmental, technical, economic and socioeconomic characteristics of each alternative; | ||
+ | *An assessment of the sensitivity of the decision making process. | ||
+ | An assessment of candidate Gunnar remedial options was conducted in general accordance with the | ||
+ | framework for MCDA provided by Ref. [124], which is provided as a case study. | ||
+ | |||
+ | =====''Remedial options analysis''===== | ||
+ | A description of the key steps in remedial options analysis (see Table 23) was developed by Environment | ||
+ | Canada in 2011 [124]. This approach has been applied to evaluate unfiltered candidate options for remediation at | ||
+ | the Gunnar Mine Site.<br> | ||
+ | ''<big>Step 1: Identification of candidate alternatives</big>''<br> | ||
+ | A first step in the assessment of remedial options is to develop a list of all possible candidate remedial | ||
+ | options, with input from stakeholders and without prejudgement, i.e. to generate an ‘unfiltered’ list of remedial | ||
+ | options [124, 125]. In doing so, a basic set of ‘threshold criteria’ (or ‘exclusion criteria’) may be established that | ||
+ | can then be used in the prescreening of unfiltered options, as part of step 2 of the remedial options analysis.<br> | ||
+ | For the purposes of options assessment, candidate alternatives were identified using a two phased approach: | ||
+ | compilation of an unfiltered list of remedial options and then the evaluation of this list. The unfiltered list of | ||
+ | remedial options that was generated during the first phase was distributed for review and input to responsible | ||
+ | authorities, including provincial and federal regulatory and project partner stakeholder groups, in the second phase. | ||
+ | The unfiltered remediation alternatives were generated to address each of the following Gunnar Site components, | ||
+ | which were identified in the Gunnar EIA as the major ‘aspects’ of the site remediation: | ||
+ | *Waste disposal (i.e. disposal of waste generated by the demolition of former site infrastructure); | ||
+ | *Waste rock deposit; | ||
+ | *Open pit; | ||
+ | *Hydrocarbon contaminated soil; | ||
+ | *Waste rock seepage; | ||
+ | *Groundwater seepage; | ||
+ | *Gunnar main tailings (including tailings downstream of the dyke at the eastern boundary of the Gunnar main tailings deposit); | ||
+ | *Gunnar central tailings; | ||
+ | *Langley Bay/Back Bay (including tailings and aquatic receiving environment). | ||
+ | The candidate alternatives that were generated through this exercise constituted a broad list of waste disposal | ||
+ | technologies, storage options and disposal locations, both on-site and off-site.<br> | ||
+ | ''<big>Step 2: Prescreening assessment</big>''<br> | ||
+ | Following identification of the candidate remediation alternatives (as part of step 1 above), a prescreening | ||
+ | assessment of the unfiltered list was conducted, based on threshold criteria (or exclusion criteria). The objective of | ||
+ | the prescreening was to optimize the decision making process, with focus on an appropriate and manageable set of | ||
+ | sufficiently detailed alternatives, as opposed to evaluating options that have obvious deficiencies [124].<br> | ||
+ | Once the generic alternatives had been identified, based on reason, practicality and precedence, any ‘fatal | ||
+ | flaw’ for each alternative was identified via preliminary screening. The screening of options was performed against | ||
+ | threshold/exclusion criteria specific to the project objectives/desired end points. These criteria were established so | ||
+ | as to generate a simple ‘yes’ or ‘no’ answer, dictating which alternatives were to be carried through to more detailed | ||
+ | analysis. The established threshold (or exclusion) criteria were as follows: | ||
+ | *Does the alternative allow for compliance with a total external gamma dose criteria (mean value of ≤ 1 μSv/habove the background at a 1 m height, averaged over a 100 m × 100 m area and maximum value of 2.5 μSv/habove the background at a 1 m height, based on close out criteria for Cluff Lake, a uranium mine/mill sitein northern Saskatchewan further along in the decommissioning/remediation process than Gunnar [126])(Gunnar background = 0.7 μSv/h)? (Answer of ‘no’ indicates a fatal flaw.) | ||
+ | *Does the alternative allow for long term compliance with site specific/Saskatchewan Environment/Canadian Council of Ministers of the Environment guidelines/objectives/standards for soil and surface water quality? (Answer of ‘no’ indicates a fatal flaw.) | ||
+ | *Does the alternative allow for compliance with pertinent provincial/federal legislation? (Answer of ‘no’ indicates a fatal flaw.) | ||
+ | *Is the alternative a proven technology/has it been completed elsewhere with successful results? (Answer of ‘no’ indicates a fatal flaw.) | ||
+ | *Does the alternative improve the level of risk at the site? (Answer of ‘no’ indicates a fatal flaw due to an increase in risk.) | ||
+ | *Is there a potential risk of catastrophic failure? (Answer of ‘yes’ indicates a fatal flaw.) | ||
+ | *For waste disposal, is the proposed disposal site sufficiently large to accommodate waste? (Answer of ‘no’ indicates a fatal flaw.) | ||
+ | Each alternative receiving a positive evaluation (i.e. with no fatal flaws identified) was carried through a | ||
+ | multiple accounts analysis (as demonstrated in Table 20), where the impacts from each alternative were assessed | ||
+ | through a transparent scoring and weighting process.<br> | ||
+ | ''<big>Step 3: Characterization of alternatives</big>''<br> | ||
+ | Once the prescreening assessment of candidate remedial alternatives had been completed, the remaining | ||
+ | options required characterization. During options characterization, the options for each major site element were | ||
+ | evaluated by a multidisciplinary project team consisting of individuals with expertise in the natural sciences, | ||
+ | contaminant dynamics and engineering. Only potentially feasible alternatives were carried through to this stage | ||
+ | of analysis. Feasible alternatives included those that could represent implementable remedial options, with no | ||
+ | fatal flaws being identified during prescreening with respect to project objectives and end points. For clarity and | ||
+ | differentiation from prescreening of remedial ‘alternatives’, potentially feasible alternatives that were carried | ||
+ | through to detailed analysis were renamed as ‘options’ (as opposed to alternatives).<br> | ||
+ | The outcomes of the evaluations of HHERA were derived in the context of the following broad evaluation | ||
+ | criteria, which were developed for the site as part of the Gunnar EIA: | ||
+ | *Human health risks; | ||
+ | *Terrestrial wildlife and vegetation; | ||
+ | *Aquatic life and vegetation. | ||
+ | Within each of the above broad criteria, more detailed evaluation criteria (or ‘subaccounts’) were established, | ||
+ | as described under step 4 below.<br> | ||
+ | The focus of the evaluation of options with respect to HHERA was centred around potential option related | ||
+ | chemical and radiological impacts to members of the public and the environment. In addition, physical impacts | ||
+ | to terrestrial and aquatic life and vegetation were considered as part of the ecological risk assessment related | ||
+ | evaluation criteria. As the Gunnar Mine Site has been secured and is not open to the public without orientation, and | ||
+ | work activities are being planned and implemented to protect people, physical impacts to humans were considered | ||
+ | as part of the evaluation of constructability.<br> | ||
+ | The constructability evaluation was focused on the following two evaluation criteria: | ||
+ | *Potential risks to humans (i.e. workers) and the environment during the execution of the remediation (as part of the ‘active remediation phase’); | ||
+ | *Constructability/feasibility/efficacy of a given remedial option from a ‘practicability of execution’ perspective. | ||
+ | Following evaluation of remedial options with respect to HHERA and constructability, further screening was | ||
+ | conducted to identify the potentially acceptable options based on public preference, gained through engagement | ||
+ | of local communities and leaders, both through providing residents with information on the project, and receiving | ||
+ | feedback and advice from communities. This was accomplished through community meetings, surveys and | ||
+ | other forums, which provided important insight with respect to public preferences regarding the planning and | ||
+ | implementation of the project, and which were considered in the evaluation of the Gunnar remedial options.<br> | ||
+ | ''<big>Step 4: Multiple accounts ledger</big>''<br> | ||
+ | Following identification and characterization of alternatives in steps 1–3, it was necessary to then evaluate the | ||
+ | options that were identified as potentially feasible, by identifying elements that could be used to differentiate them. | ||
+ | This was accomplished through the development of a multiple accounts ledger, which consisted of the following | ||
+ | two elements: subaccounts (also called evaluation criteria) and indicators (also called measurement criteria). | ||
+ | Subaccounts (or evaluation criteria) were developed using the characterization criteria that had been selected | ||
+ | during step 3. Unlike the characterization criteria, which were factual criteria that had been assigned with no a | ||
+ | priori judgement being made regarding any of the alternatives under consideration, the evaluation criteria were | ||
+ | developed with consideration of the material impact (benefit or loss) associated with each of the options under | ||
+ | evaluation.<br> | ||
+ | Evaluation criteria with respect to the Gunnar remediation project were, therefore, set in the context of three | ||
+ | broad categories (or ‘accounts’): HHERA, constructability and public preference, as described above.<br> | ||
+ | Within the above evaluation criteria, a number of qualitative and quantitative measurement criteria were | ||
+ | established, which were then scored to identify potentially feasible remedial options.<br> | ||
+ | The following measurement criteria were identified with respect to potential worker and ecological health | ||
+ | risks during remediation: | ||
+ | *Physical hazards (safety risks); | ||
+ | *Worker health risks via exposure to non-radiological and radiological contaminants (soil or dust inhalation, dermal contact), ambient radon exposure and external radiation exposure; | ||
+ | *Risks to terrestrial wildlife generated; | ||
+ | *Risks to aquatic life generated. | ||
+ | The following measurement criteria were identified with respect to constructability, feasibility and efficacy | ||
+ | during remediation: | ||
+ | *Estimated construction duration; | ||
+ | *Cost; | ||
+ | *Ability to mitigate pertinent exposure pathways; | ||
+ | *Regulatory acceptance; | ||
+ | *Long term waste generation; | ||
+ | *Requirement for control, monitoring and/or maintenance (i.e. institutional control). | ||
+ | The possible Gunnar remedial options were evaluated in the context of a single integrated measurement | ||
+ | criterion that has been identified as ‘public preference’, which was scored as described in further detail below.<br> | ||
+ | ''<big>Step 5: Value based decision process</big>''<br> | ||
+ | Once a multiple accounts ledger had been created, with criteria to facilitate the evaluation and measurement | ||
+ | of possible remedial options, it was necessary to score and weight all the indicators, subaccounts and accounts, | ||
+ | such that merit ratings for each option could be quantitatively determined. In doing so, the following processes | ||
+ | were undertaken: | ||
+ | *Scoring; | ||
+ | *Weighting; | ||
+ | *Quantitative analysis. | ||
+ | Scoring of the possible Gunnar remedial options from the perspective of HHERA in the long term was | ||
+ | conducted using both quantitative and qualitative measurement criteria. Quantitative criteria were based on | ||
+ | the concentrations of contaminants released to the environment in the long term for a given option relative to | ||
+ | environmental quality guidelines, whereas qualitative criteria provided a relative comparison of remedial options in | ||
+ | cases for which no quantitative guidelines existed against which measurement criteria could be compared. On this | ||
+ | basis, the following scoring criteria were developed: | ||
+ | *A value of 0 indicates exceedance of the guideline by 100-fold or greater/physical risk that exceeds the baseline risk; this option performs very poorly against listed criteria. | ||
+ | *A value of 1 indicates exceedance of the guideline by 10- to 100-fold/physical risk that is similar to the baseline risk; this option is the same or has a very small improvement compared with the existing situation. | ||
+ | *A value of 2 indicates exceedance of the guideline by 1- to 10-fold/physical risk that is lower than the baseline risk; this option has a moderate improvement compared with the existing situation. | ||
+ | *A value of 3 indicates not applicable; this option has no exceedance of guideline values or a large improvement compared with the existing situation/best performing option. | ||
+ | To differentiate the performance of the options versus the prescribed criteria, numerical rankings were | ||
+ | assigned as a quantitative indicator of impact. The following measurement scheme was used with respect to | ||
+ | constructability: | ||
+ | *A value of 0 indicates the option performs very poorly against listed criteria. | ||
+ | *A value of 1 indicates the same or a very small improvement compared with the existing situation. | ||
+ | *A value of 2 indicates moderate improvement compared with the existing situation. | ||
+ | *A value of 3 indicates not applicable/large improvement compared with the existing situation/best performing option. | ||
+ | In terms of options that were ‘do nothing’ or very near to do nothing, many of the criteria in the technical | ||
+ | analysis were evaluated as ‘not applicable’. These not applicable designations were given a ranking of 3, to indicate | ||
+ | a high ranking in terms of subaccounts, such as duration or cost, in comparison with options for which some action | ||
+ | is taken.<br> | ||
+ | Scoring of each remedial option with respect to public preference was undertaken using an integrated approach | ||
+ | to incorporate information that had been received from community members and leaders through a diversity of | ||
+ | consultation approaches. The outcomes of such approaches, which included community meetings, flyers, surveys | ||
+ | and many others, were used to inform the scoring in the context of the following scoring criteria: | ||
+ | *A value of 0 indicates this option is unacceptable or that local communities are opposed to the option. | ||
+ | *A value of 1 indicates local communities would consider it as a possible option, but with reservations. | ||
+ | *A value of 2 indicates local communities would consider it as a possible option. | ||
+ | *A value of 3 indicates local communities would consider it as a preferred option. | ||
+ | It should be noted that in assigning scores of 1 versus 2 above, values were set to distinguish options that | ||
+ | were considered possible by local communities, in one case, with reservations, and in the second case, with few | ||
+ | to no reservations. For example, a score of 1 was assigned for options involving transport of hazardous materials | ||
+ | across Lake Athabasca. In such scenarios, it was necessary for activities to be conducted in close consultation with | ||
+ | communities owing to the value that northern communities place on water quality and the concerns of catastrophic | ||
+ | releases of hazardous materials to Lake Athabasca. Despite these reservations, it was possible to transport hazardous | ||
+ | materials across the winter ice road, working closely with local communities.<br> | ||
+ | As part of the analysis, weighting factors were applied to the raw scores that had been generated during the | ||
+ | evaluation of possible Gunnar remedial options. In this way, it was possible to assign a relative importance of a | ||
+ | given measurement criterion compared to others.<br> | ||
+ | As a preliminary step, weighting factors were assigned to each of the three accounts (HHERA, constructability | ||
+ | and public preference), as follows: | ||
+ | *A value of 40% for HHERA; | ||
+ | *A value of 40% for constructability; | ||
+ | *A value 20% for public preference. | ||
+ | Within each of the above three accounts, individual measurement criteria were also weighted and justified to | ||
+ | reflect their relative importance.<br> | ||
+ | Once scoring and weighting of each possible remedial option was completed, it was necessary to conduct a | ||
+ | quantitative analysis of the information. This involved compiling the indicator value ''S'' (‘score’) for each indicator | ||
+ | (or measurement criterion), along with the weighting factor ''W'', and calculating the product of these to generate a | ||
+ | combined indicator merit score (''S·W'') for each possible remedial option [124].<br> | ||
+ | Based on these data, it was possible to take the sum of the indicator merit scores for each evaluation criterion | ||
+ | (or subaccount) to generate a subaccount merit score for each, i.e. ''Σ{S·W}''. Within a given subaccount (or evaluation | ||
+ | criterion), the resultant subaccount merit scores could then be directly compared across possible remedial options. | ||
+ | To facilitate further comparison of each subaccount merit score against values for other subaccounts, the | ||
+ | scores were normalized to the same point scale used to score each indicator value. In doing so, the subaccount | ||
+ | merit score was divided by the sum of the weightings (Σ W) to yield a subaccount merit rating [124]:<br> | ||
+ | <center><math>R_S=\frac{\sum{S\cdot W}}{\sum{W}}</math>; (7)</center> | ||
+ | The same procedure can be undertaken to weight and normalize scoring for accounts (in this case, HHERA, | ||
+ | constructability and public preference) to generate account merit scores (''Σ{Rs·W}'') and account merit ratings | ||
+ | (''Σ{Rs·W}/ΣW'') for each remedial option.<br> | ||
+ | As a final step, option merit scores (''Σ{Ra·W}'') and option merit ratings (''Σ{Ra·W}/ΣW'') can be determined for | ||
+ | each possible Gunnar remedial option.<br> | ||
+ | It should be noted that even with the multiple layers of analysis, it is possible for certain options to score | ||
+ | identically, against all criteria, for a certain aspect of the site. In the event of such scoring/ranking, the performance | ||
+ | of the option needs to be measured against the total available score, as described below.<br> | ||
+ | ''<big>Step 6: Sensitivity analysis''</big><br> | ||
+ | Any decision making process will be inherently subject to some degree of bias and subjectivity. Therefore, | ||
+ | it is important that a framework is followed to ensure that any decisions arising from the options screening and | ||
+ | selection process are transparent and reproducible by external reviewers [124]. With this in mind, the options | ||
+ | analysis was completed for the Gunnar Mine Site in multiple tables, rating the potential remedial options separately | ||
+ | with respect to performance against: (i) HHERA, (ii) constructability and (iii) public preference, to allow for such | ||
+ | transparency.<br> | ||
+ | A sensitivity analysis can involve a very large number of permutations and combinations, which in itself can | ||
+ | impose bias and subjectivity. Therefore, for the purposes of the Gunnar evaluation, the sensitivity analysis was | ||
+ | kept simple, with the ultimate goal of evaluating the impact of the respective indicator weights (W) on the selection | ||
+ | of potentially feasible remedial options, based on the initial ranking (i.e. assignment of a value of 0–3 for each | ||
+ | measurement criterion and remedial option combination). As such, the analysis was conducted by simply setting all | ||
+ | weightings to a value of 1, effectively removing the value bias between one measurement criterion and another. In | ||
+ | this case, remedial options achieving relatively higher sums of indicator values measured against the measurement | ||
+ | criterion values may be more feasible for a given site aspect than those with lower indicator values. A sensitivity | ||
+ | analysis was conducted to evaluate this. | ||
+ | |||
+ | =====''Summary of the study results''===== | ||
+ | An assessment of candidate remedial options was conducted in general accordance with the framework for | ||
+ | MCDA provided by Ref. [124]. The analysis consisted of multiple stages of analysis, as demonstrated in Table 23. | ||
+ | Based on this approach, a comprehensive, unfiltered list of possible remedial options for the Gunnar Mine Site was | ||
+ | evaluated and scored to identify potentially feasible remedial options. | ||
+ | For the purposes of this analysis, a potentially preferable option was identified as the option for which the | ||
+ | highest option merit rating was determined. Owing to the inherent complexity of the Gunnar remediation project, | ||
+ | it was necessary to subdivide remedial options into a number of interconnected site aspects (e.g. submerged and | ||
+ | unsubmerged unconfined tailings, waste rock piles, open pit, etc.), which were individually scored as separate | ||
+ | matrices, with respect to HHERA and public preference.<br> | ||
+ | Based on this analysis, potentially preferable remedial options were identified to address each Gunnar Site | ||
+ | aspect; however, due to the lack of historical records and monitoring data, it was not possible to identify a single, | ||
+ | ‘preferred’ remedial option. Instead, potential remedial options could be subdivided into three categories [127]:<br> | ||
+ | (a) Options that are relatively straightforward to implement, with little uncertainty, e.g. covering unconfined | ||
+ | tailings, water diversions, removal of contaminated debris from waste rock piles and addressing physical | ||
+ | hazards associated with unstable slopes on the waste rock piles.<br> | ||
+ | (b) Options that require additional monitoring data and follow-up assessment prior to selection of a final | ||
+ | remediation approach, e.g. further surface water and hydrogeological monitoring to determine contaminant | ||
+ | transport pathways between sources and receiving environments.<br> | ||
+ | (c) Options that are dependent upon the selection of remedial approaches for other site aspects that have to be | ||
+ | determined before the first one can proceed, e.g. decisions on the pit cannot be determined until it has been | ||
+ | decided if the waste rock will be placed in the pit or covered and left in place. | ||
+ | |||
+ | ==Appendix IV== | ||
+ | <big>'''Concept of collective dose'''</big><br> | ||
+ | The concept of collective dose was found to be very useful for comparative analysis of radioactive emissions | ||
+ | from nuclear facilities and systems. | ||
+ | |||
+ | ===Introduction=== | ||
+ | Interpretation of the collective dose has been discussed by the ICRP, which, in its recommendations [128], | ||
+ | believes that the use of the collective dose concept is closely connected with formal CBA. The product of the | ||
+ | average dose to an individual and the number of individuals in a group (i.e. how collective dose has been calculated | ||
+ | in the past) is a legalized quantitative value, but it is of a limited application, as it combines redundant information. | ||
+ | For decision making, the necessary information has to be presented as a matrix that indicates the number of | ||
+ | individuals exposed to a specific dose and the date it was received. This matrix needs to be considered as an | ||
+ | auxiliary decision making tool allowing the significance evaluation of individual matrix elements. The matrix | ||
+ | approach leads to a more correct estimation of consequences (risks) of irradiation. The ICRP believes that this | ||
+ | will avoid misinterpretation of the collective dose, which has resulted in serious errors in the prediction of lethal | ||
+ | outcomes. A justification of these ICRP recommendations can be found in Ref. [128].<br> | ||
+ | Collective doses are generally not used by regulators; instead, regulators usually limit the emission of single | ||
+ | radionuclides or classes of radionuclides represented by equivalent isotopes on the basis of doses to the critical | ||
+ | group. However, collective doses normalized per unit of electricity were used in the ExternE project for the | ||
+ | calculation of external costs for comparing different power technologies [110, 111, 119]. Annual collective doses | ||
+ | have also been used for comparison of different options of nuclear cycles in Ref. [116]. Recommendations on how | ||
+ | collective doses should be calculated in particular cases can be found in Refs [23, 129, 130].<br> | ||
+ | Use of collective doses also presents some disadvantages, e.g. the possibly prevailing importance given to | ||
+ | very low doses occurring over very long time periods (applying the linear dose effect relationship without cut-offs), | ||
+ | the dependency on key modelling assumptions that are not easily controllable such as population distributions | ||
+ | (in place and time) and the application, or not, of discounting. | ||
+ | |||
+ | ===Simplified determination of collective dose=== | ||
+ | The IAEA generic models (see also Appendix II) include a simplified method to determine collective doses. | ||
+ | In section 7 of Ref. [23], tables contain collective effective dose commitments per unit activity (man Sv/Bq) | ||
+ | of radionuclides discharged to the atmosphere, to marine water and freshwater bodies. All contributions from | ||
+ | individual radionuclide species and pathways need to be summed.<br> | ||
+ | The simplified conversion of release rates to dose factors has been derived using the results of two approaches: | ||
+ | one based on a simple method using generic parameters [98] and the other one based on complex modelling [131], | ||
+ | which have been developed by the NRPB, in the United Kingdom. Reference [23] suggests using simplified models | ||
+ | with caution, noting that they can only provide order of magnitude estimates, and that collective doses: | ||
+ | <blockquote> | ||
+ | “...should be used only as part of a screening or generic assessment procedure, for example to ensure | ||
+ | compliance with dose limiting criteria or as input to an optimization exercise to compare options as part of an | ||
+ | intuitive, semi-quantitative analysis. They should not be used for more rigorous optimization analyses, such | ||
+ | as cost–benefit analyses, nor for other purposes.” | ||
+ | </blockquote> | ||
+ | Reference [23] further states that “the site specific discharge conditions and the actual critical group location | ||
+ | be taken into account if the predicted doses exceed a reference level of around 10% of the dose constraint.”<br> | ||
+ | Collective doses were estimated for most radionuclides only in local and regional zones, which may extend | ||
+ | from the point of release to distances varying from about a hundred kilometres to several thousand kilometres [23]. | ||
+ | Four nuclides were considered for global analysis because of their relatively long radioactive half-lives or a high | ||
+ | environmental mobility: <sup>14</sup>C, <sup>3</sup>H, <sup>129</sup>I and <sup>85</sup>Kr; other long lived radionuclides, such as <sup>237</sup>Np or <sup>99</sup>Tc, may also | ||
+ | become globally dispersed following discharge, but they have not yet been accurately addressed. Therefore, | ||
+ | an analyst may need to consider these (and others), depending on the technical characteristics and expected | ||
+ | performance of the NES.<br> | ||
+ | Collective doses can be used for the purposes of optimization of radiation protection measures. WS-G-2.3 [33] | ||
+ | recommends using the estimations of collective dose arising from discharges to avoid spending resources to assess | ||
+ | options for reducing discharges in disproportion to the likely improvement in radiological protection. The collective | ||
+ | dose from discharges, which can be estimated using Ref. [23], should be added to an estimate of the relevant | ||
+ | collective dose from occupational exposure to provide an estimate of the total collective dose. If the result is less | ||
+ | than about 1 man Sv/a, an extensive formal optimization study most probably will not be needed [132]. If the value | ||
+ | of the collective dose is greater than about 1 man Sv/a, a formal study by the designer (technology developer) is | ||
+ | required, with the use of decision aiding techniques such as CBA and multicriteria methods. |
Revision as of 09:51, 30 July 2020
INPRO basic principle in the area of environmental impact of stressors: The expected adverse environmental
effects of an NES should be well within the performance envelope of current NESs delivering similar energy products.
Contents
- 1 Introduction
- 2 General features of an environmental assessment
- 3 Necessary INPUT for an INPRO assessment in the area of environmental impact of stressors
- 4 INPRO basic principle, user requirements and criteria in the area of environmental impact of stressors
- 4.1 INPRO basic principle: acceptability of expected adverse environmental effects
- 4.2 User requirement UR1: controllability of environmental stressors
- 4.3 User requirement UR2: reduction of total environmental impact of emitted radioactivity
- 4.4 User requirement UR3: optimization of the measures to reduce environmental impact
- 5 Appendix I
- 5.1 Introduction
- 5.2 Mining of uranium ore
- 5.3 Milling of uranium ore
- 5.4 Refining and conversion of uranium ore to uranium hexafluoride
- 5.5 Enrichment of uranium
- 5.6 Conversion to uranium dioxide and fuel fabrication
- 5.7 Mixed oxide fuel fabrication
- 5.8 Power generation
- 5.9 Reprocessing
- 5.10 Storage of spent nuclear fuel away from the reactor
- 5.11 Disposal of radioactive waste
- 6 Appendix II
- 6.1 Introduction
- 6.2 IAEA generic models for radiological impact on humans
- 6.3 Concepts, models and tools for assessment of radiological impact on non-human biota
- 6.3.1 International Commission on Radiological Protection approach
- 6.3.2 Environmental Risk from Ionising Contaminants: Assessment and Management tool
- 6.3.3 Department of Energy graded approach and RESRAD-BIOTA tool
- 6.3.4 Limits for radiation protection of non-human biota and their application for environmental assessments
- 6.4 International activities related to radiological impact on non-human biota
- 6.5 Calculation of impacts of chemical stressors on humans and the environment
- 6.6 Representative examples of environmental studies
- 7 Appendix III
- 8 Appendix IV
Introduction
Objective
This volume of the updated INPRO Manual provides guidance to the assessor of an NES (or a facility thereof)
that is planned to be installed, describing how to apply the INPRO methodology in the area of environmental impact
of stressors. The INPRO assessment should either confirm the fulfilment of all INPRO methodology environmental
CRs, or identify gaps (non-compliance with the INPRO methodology CRs) requiring corrective actions (including
research, development and demonstration (RD&D)) to achieve long term sustainability of the NES assessed.
The INPRO assessor (or team of assessors) is assumed to be knowledgeable in the area of environmental
impact of stressors and/or may be using the support of qualified national or international organizations
(e.g. the IAEA) with relevant experience.
Two general types of INPRO assessor can be distinguished: a nuclear technology holder (i.e. designer,
developer or supplier of nuclear technology) and a (potential) user of such technology. The role of the latter type of
assessor, i.e. a technology user, is primarily to check, in a simplified manner, whether the designer (supplier) has
appropriately taken into account the environmental aspects in the design as defined by the INPRO methodology.
A technology user is assumed to be primarily interested in proven technology to be installed in his or her country in
the near future. A designer (developer) performing an INPRO assessment can use this current publication to check
whether the (innovative) design under development, which is expected to comply with the existing IAEA safety
standards, meets the INPRO methodology environmental requirements for the assessment of sustainability of NES,
and can additionally initiate modifications during early design stages, if necessary, to improve the environmental
performance of the design.
In INPRO sustainability assessment, the term ‘technology user’ does not refer to an independent national
nuclear regulatory authority. INPRO sustainability assessment is most often part of strategic nuclear energy
planning. Typically, this planning activity is performed by national energy ministries, utilities (government or
privately held) and/or their various technical support organizations. In INPRO terminology, the ‘technology user’
is either the country (in a generic sense) or, more specifically, the owner and/or operator of the technology. The
‘technology holder or designer’ is the engineering company (government or privately held) that holds the intellectual
property rights on the technology. When discussing the specific context where trade agreements between countries
restrict or control transfers and uses of technology, the INPRO use of the term ‘technology holder’ may also refer to
the government under which the technology is ‘flagged’.
An assessor in a country embarking on a nuclear power programme has several options when using the
INPRO methodology that depend on the stage of the programme (see the introductory manual of the updated
INPRO methodology).
Every nuclear facility needs an EIA in order to be licensed and to become operational. The INPRO
environmental assessment is not intended to substitute this licensing activity, but should rather demonstrate that the
NES assessed is sustainable in the long term with regard to its environmental impact. Application of the INPRO
methodology cannot substitute fulfilment of any of the existing national requirements in this area. However, INPRO
CR determined in this publication follow the requirements of the IAEA safety standards, in particular, IAEA Safety
Standards Series No. GSR Part 3, Radiation Protection and Safety of Radiation Sources: International Basic Safety
Standards [2].
Scope
Environmental impact from NES involves two large groups of factors. One group impacting the environment
comprises the consumption of non-renewable resources including both fissile/fertile materials necessary to produce
nuclear fuel and other materials (e.g. zirconium). All these factors and consumption of electricity necessary to
construct, operate and occasionally decommission NES installations are considered in the INPRO methodology
manual on environmental impact from depletion of resources [3].
Another group comprises radiological, chemical, thermal and other stressors which NESs release into
environment. This group also includes water intake because this factor can be important for biota even when this
water is returned to the environment in a clean form (e.g. as steam from nuclear power plant cooling towers). All
these factors are considered in this INPRO methodology manual on environmental impact of stressors.
The INPRO methodology in the area of environmental impact of stressors covers only normal operation
and anticipated operational occurrences of NES facilities. Consequences of potential accidents are discussed in
the INPRO methodology manuals in the areas of safety of reactors and safety of the nuclear fuel cycle. Guidance
provided here, describing good practices, represents expert opinion but does not constitute recommendations made
on the basis of a consensus of Member States
Structure
In Section 2, general features of an environmental assessment are presented.
In Section 3, an overview of information that must be available to an INPRO assessor to perform the
environmental assessment is provided.
In Section 4, the background of the INPRO methodology BP for environmental impact of stressors, and the
corresponding URs and CRs, consisting of INs and ALs, are presented. At the CR level, guidance is provided on
how to determine the values of the INs and ALs.
Appendix I provides general information on types of stressor and separate, illustrative lists of stressors
(in the form of tables) for all facilities of an NES based on uranium and mixed oxide (MOX) fuel (as an example).
This appendix could be used by an INPRO assessor as a starting point to generate input for the BP evaluation.
Appendix II presents simplified environmental analysis methods of how to calculate the impact of radiological
stressors, i.e. the dose on humans and non-human biota (plants and animals). It also briefly discusses the calculation
of the impacts of chemical stressors on humans and non-human biota.
Appendix III illustrates the concepts for optimization of the management options for reduction of the
environmental impact of nuclear facilities.
Appendix IV provides basic information on the concept of collective dose, which is used in the INPRO
assessment method described in this publication.
Table 1 provides an overview of the BP, URs and CR in the INPRO methodology area of environmental
impact of stressors.
INPRO basic principle the area of environmental impact of stressors (acceptability of expected adverse environmental effects): The expected adverse environmental effects of an NES should be well within the performance envelope of current NESs delivering similar energy products. | ||
INPRO user requirements | Criteria | Indicator (IN) and Acceptance Limit (AL) |
---|---|---|
UR1: Controllability of environmental stressors: The environmental stressors from each facility of an NES over the complete life cycle should be controllable to levels meeting or below current standards
|
CR1.1: Radiation exposure of the public | IN1.1: Dose to the public |
AL1.1: Lower than the dose constraint | ||
CR1.2: Radiation exposure of non-human species | IN1.2: Doses to the reference biota species | |
AL1.2: Lower than international recommendations | ||
CR1.3: Impacts of chemicals and other non-radiation environmental stressors | IN1.3: Levels of chemicals and other stressors | |
AL1.3: Lower than national environmental safety standard levels | ||
UR2: Reduction of total environmental impact of emitted radioactivity:
Total radiotoxicity of radionuclides discharged by the NES assessed should be lower than that of any current NES delivering similar energy products |
CR2.1: Reduction of environmental impact of radiation | IN2.1: Total radiotoxicity of radionuclides emitted to the environment from the NES assessed (RT) |
AL2.1: RT is lower than the radiotoxicity of stressors emitted to the environment from a current NES delivering similar energy products | ||
UR3: Optimization of the measures to reduce environmental impact:
The measures applied to reduce adverse environmental impact attributable to an NES should be optimized |
CR3.1: Optimization of the measures to reduce environmental impact | IN3.1: Measures to reduce environmental impact of the NES |
AL3.1: Measures are optimized |
Concept of sustainable development and its relationship with the area of environmental impact of stressors of the INPRO methodology
The United Nations World Commission on Environment and Development Report [4] (often known as the Brundtland Report), entitled Our Common Future, defines sustainable development as: “development that meets the needs of the present without compromising the ability of future generations to meet their own needs” (see chapter 2, para. 1 [4]). This definition contains within it two key concepts:
“
- the concept of ‘needs’, in particular the essential needs of the world’s poor, to which overriding priority should be given; and
- the idea of limitations imposed by the state of technology and social organization on the environment’s ability to meet present and future needs.”
Based on this definition of sustainable development, a three part test for any approach to sustainability and
sustainable development was proposed within INPRO project: (i) current development should be fit for the purpose
of meeting current needs with minimized environmental impacts and acceptable economics; (ii) current RD&D
programmes should establish and maintain trends that lead to technological and institutional developments that
serve as a platform for future generations to meet their needs; and (iii) the approach to meeting current needs
should not compromise the ability of future generations to meet their needs.
At first reading, this definition may appear obvious, but when considering the complexities of implemented
nuclear energy technology and systems, plus their many supporting institutions, meeting the three part test is not
always straightforward because many approaches only meet one or perhaps two parts of the test in a given area,
and may fail on the others.
The Brundtland Report overview (para. 61 [4]) of the topic of nuclear energy summarized that:
“After almost four decades of immense technological effort, nuclear energy has become widely used. During this period, however, the nature of its costs, risks, and benefits have become more evident and the subject of sharp controversy. Different countries world-wide take up different positions on the use of nuclear energy. The discussion in the Commission also reflected these different views and positions. Yet all agreed that the generation of nuclear power is only justifiable if there are solid solutions to the unsolved problems to which it gives rise. The highest priority should be accorded to research and development on environmentally sound and ecologically viable alternatives, as well as on means of increasing the safety of nuclear energy.”
The Brundtland Report presented its comments on nuclear energy in chapter 7, section III [4]. In the area of
nuclear energy, the focus of sustainability and sustainable development is on solving certain well known problems
(referred to here as ‘key issues’) of institutional and technological significance. Sustainable development implies
progress and solutions in the key issue areas. Seven key issues are discussed (in this order):
(a) Proliferation risks;
(b) Economics;
(c) Health and environment risks;
(d) Nuclear accident risks;
(e) Radioactive waste disposal;
(f) Sufficiency of national and international institutions (with particular emphasis on intergenerational and
transnational responsibilities);
(g) Public acceptability.
The INPRO methodology for the self-assessment of sustainability and sustainable development of an NES is
based on the broad philosophical outlines of the Brundtland Report’s concept of sustainable development described
above. Twenty-nine years have passed since the publication of the Brundtland Report, and 15 years have passed
since the initial consultancies on development of the INPRO methodology in 2001. In the interim period of time,
significant historical events have starkly highlighted certain key issues. However, the key issues for sustainable
development of NESs have remained essentially unchanged over nearly three decades.
By far the most notable events in the period, which have a direct impact on nuclear energy sustainability, are
related to non-proliferation, nuclear security, cost escalation of new construction and, most notably, the accident at
the Fukushima Daiichi nuclear power plant in 2011. The Fukushima Daiichi accident further clarified that nuclear
safety is an issue of paramount importance for sustainability and that external hazards, associated with a particular
site, could be responsible for a dramatic common cause failure involving multiple reactor units.
In each INPRO methodology manual, a key issue of NES sustainable development is examined. The structure
of the methodology is a hierarchy of INPRO BPs, URs and CRs measuring whether the UR has been achieved.
Under each BP, the CRs include measures that take into consideration the three part test based on Brundtland
Report’s definition of sustainable development which was described above.
This INPRO Manual focuses on the key issue of environmental stressors (radioactive, chemical, heat and
others) associated with NES development and deployment. It does not consider emissions of environmental
stressors under accident conditions. The topics of radioactive and chemical emissions during accidents are covered
under the INPRO areas of safety of reactors and fuel cycle facilities and are published in separate manuals.
In the broad sense of the full related technology chain, NESs are comparatively benign with respect to many
stressor emissions when compared to available, baseload non-NESs. For example, because the waste products
of nuclear fission are contained during all but severe nuclear accidents, releases of radiological species to the
environment from operating nuclear reactors are typically extremely small — at least one and often two or more
orders of magnitude below national regulatory limits and international standards [5]. In fact, radioactive emissions
from coal fired thermal power plants are often higher than for nuclear reactors of comparable scale. This is because
the coal and the resulting fly ash waste contain uranium and thorium oxides and radioactive progenies that are not
fully captured by emission controls. Chemical stressor emissions from nuclear reactors are also very small, whereas
chemical stressors (sulphur oxides, nitrogen oxides (NOX), mercury, dioxins, volatile aromatic hydrocarbons, etc.)
can be significant fugitive components of emissions from coal fired power plants. Although far cleaner than coal,
natural gas fired plants contribute significant emissions of NOX and emissions of methane associated with fuel
leaks. If carbon emissions are considered as an environmental stressor (an increasingly common position globally),
nuclear power is benign and comparable to renewable energy power sources such as hydroelectricity.
Typically, the largest shares of radiological and chemical stressor emissions from NESs originate from
mining, milling and spent fuel reprocessing fuel cycle facilities, as opposed to from nuclear reactors.
One area where nuclear power has similar environmental stressor impacts to other fossil fuel generation
technologies is waste heat rejection. More recently, advances such as supercritical coal and combined cycle natural
gas plants have increased thermal efficiency and thereby reduced the waste heat impacts and cooling water use
per unit of electricity produced by fossil fuel generation technology. However, if evaporative cooling towers are
used, these impacts can often be mitigated in both nuclear and fossil fuel generation technologies. Next generation
nuclear reactors also promise significant increases in thermal efficiency, and certain high temperature plant designs
may allow practical air cooling.
This current INPRO Manual tests whether an NES is sustainable with respect to environmental stressors.
In the case of each NES installation, radiation exposure of the public is compared to the dose constraints. Radiation
exposure of non-human species is compared to the lowest values of international standards or national regulations
(when available). The total radiotoxicity of emissions is also evaluated as a broad metric to consider the reduction
of radiological impact on the environment. Moreover, the manual requests, in UR3, that evidence of optimization
of measures to reduce environmental impact is provided. Suggested optimization approaches include best available
techniques (BATs), best environmental practice (BEP), as low as reasonably achievable (ALARA) or as low as
reasonably practicable (ALARP), with social and economic factors taken into account.
As previously mentioned, environmental stressor impacts tend to be primarily associated with certain fuel
cycle facilities that are not broadly distributed. These facilities are often part of national economies of nuclear fuel
suppliers and service States that provide fuels and services as an export to other national economies. In certain
cases, it may be appropriate to parse available results of environmental assessments of these facilities to consider
separately the fraction of stressor emissions associated with national nuclear power generation and of exported
nuclear fuels and services. The INPRO CRs in this manual are set as total dose constraints and other types of
recommended or required thresholds. The sustainability requirements in the INPRO area of environmental impact
of stressors are fulfilled when all ALs are met, but it is also important to understand the balance of environmental
impacts accepted in exchange for domestic power generation (a broad public good) and in exchange for exported
nuclear fuel products and services (potentially a narrower or broader public good, depending upon the tax/tariff
structures associated with the industry). Understanding this balance is a central question in cost–benefit analysis
(CBA) regarding development of the national NES, which is discussed in the INPRO methodology area of
infrastructure [6].
The NES is expected to meet the three part test based on the Brundtland Report’s definition of sustainable
development if all ALs in all areas are met as outlined in the INPRO methodology.
Protection of the environment is a major consideration in the processes for approving industrial activities in
many countries. The level of societal concern for the environment internationally is clearly indicated in several
documents, in addition to the Brundtland Report [4], reflecting international consensus, notably the Rio Declaration
on sustainable development [7], i.e. the Rio Declaration on Environment and Development, Agenda 21, the Statement
on Sustainable Development of Forests, the United Nations Framework Convention on Climate Change and the
Convention on Biological Diversity. Other related documents are the United Nations resolution on institutional
arrangements for the Implementation of the Global Programme of Action for the Protection of the Marine
Environment from Land-based Activities [8], the Joint Convention on the Safety of Spent Fuel Management and
on the Safety of Radioactive Waste Management [9], the IAEA Convention on Nuclear Safety [10], the Convention
on Environmental Impact Assessment in a Transboundary Context (Espoo Convention) [11], the Convention on the
Prevention of Marine Pollution by Dumping of Wastes and Other Matter (London Convention) [12], the Convention
for the Protection of the Marine Environment of the North-East Atlantic [13] and the European Commission
recommendation on standardized information on radioactive airborne and liquid discharges into the environment
from nuclear power reactors and reprocessing plants in normal operation [14].
Legacy of the past
In some cases, the legacy of the past may still burden the civil nuclear industry (e.g. Refs [15–20]). If the
nuclear industry in general claims its sustainability, this should be achieved for all of its facilities to prevent
objective criticism that may challenge the assertion (e.g. imported uranium used in a sustainable NES should come
from a mining/milling operation that complies with environmental standards at the time that the uranium for the
NES was extracted).
General features of an environmental assessment
This section provides some general background information on environmental issues, particularly on the environmental impact of stressors caused by an NES.
Concept of sustainable development
The concept of sustainability can be considered from several related, but different, points of view:
social, economic, environmental and institutional. This publication deals with the environmental dimension of
sustainability by considering stressors providing adverse impact to the environment.
Protection of the environment is a major consideration in the processes for approving industrial activities in
many countries. The level of international societal concern for the environment is clearly indicated in publications
reflecting international consensus, notably the Brundtland Report [4], the Rio Declaration on sustainable
development [7] and the Joint Safety Convention of the IAEA [9].
The common basic idea in these publications is that the present generation should not compromise the ability
of future generations to fulfil their needs and should leave them with a healthy environment. Nuclear power should
support sustainable development by providing much needed energy with relatively low burdens on the atmosphere,
water, land and resource use. Improvement of the technology should include improvement of its environmental
aspects to a degree that is consistent with importance to society and with the potential environmental performance
of competing technologies.
Interfaces of a nuclear energy system with the environment
An example of the different components or facilities of a complete NES is presented in Fig. 1, starting with mining and processing, through to the final disposal of nuclear waste.
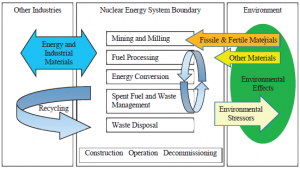
An NES has several interfaces with the environment and other industries. From the environment, non-renewable resources such as fissile and fertile materials, e.g. uranium and thorium (orange arrow in Fig. 1), are removed and used in the NES, together with other non-renewable materials such as zirconium (bright yellow arrow in Fig. 1). On the other hand, the NES releases some stressors, e.g. radioactive nuclides, that have an adverse impact on the environment (pale yellow arrow in Fig. 1). In addition to these environmental effects, an NES is exchanging with other industries energy and industrial materials required for the installation, operation and finally decommissioning of the nuclear facilities (blue arrow in Fig. 1).
Performance of an environmental analysis
Figure 2 provides a general overview of how to perform an environmental impact analysis in steps.
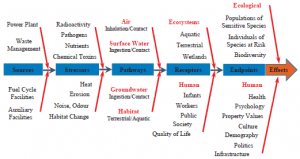
Starting from the definition of sources, i.e. the facilities of the NES, the stressors from the sources are
identified, the pathways of the stressors to the receptors analysed, leading to the end point of the environmental
analysis, i.e. the impact/effect of the stressors on the recipients. As shown in Fig. 2, Stressors of NES facilities
include radioactive and non-radioactive chemical toxic emissions, heat discharges, noise, impacts on some
non-renewable resources, and water and land use, all with potentially adverse environmental effects, which may
occur on a local, regional or even global scale.
The actual environmental effects attributable to stressors may differ significantly with geographical location
and other site specific and project specific factors of the NES facilities. However, all things being equal, the lower
the level (magnitude) of a stressor, the lower will be the resultant environmental effect. Moreover, the stressors, as
opposed to environmental pathways and receptors, are more under the control of the designers of the NES.
Comparison between nuclear and non-nuclear energy systems
The results of sustainability assessment of NESs in the area of environmental impact of stressors can be used
in comparison with other non-nuclear energy systems only when they both have been analysed to a similar depth.
For example, some environmental stressors (e.g. greenhouse gases) that may be negligible in an NES need to be
kept in the list of stressors, enabling a comparison between different sources of power generation.
Types of stressor in a nuclear energy system
Any energy system will inevitably introduce stressors to the environment, such as release of radionuclides or
non-radioactive chemicals, and depletion of resources, with potentially adverse environmental effects (or impacts)
on a local, regional or even global scale.
The environment is considered to be composed of interacting systems, which, in turn, comprise biological
elements, i.e. fauna and flora, and physical elements, i.e. atmosphere, land, water and resources (e.g. used for
energy production). Impacts are considered to be those effects that alter the existing environment, either temporarily
or permanently.
The adverse environmental impacts usually covered in an EIA as part of the site licensing process include
health effects on people and non-human species. Both radiological and non-radiological (chemical) health effects
are considered. Trade-offs and synergies among the effects from different energy system components and different
environmental stressors are also considered, if possible.
Radionuclides discharged by an NES are stressors that are specific to (but not exclusive to) nuclear power;
in many cases, the public concerns on NES safety are related to the release of radionuclides into the environment.
At the same time, there are many other stressors such as toxic chemicals (e.g. acid mine drainage from waste rock),
heat rejections from once through cooling systems, land use, etc., that, in specific cases, might have a greater
negative impact than radionuclides released into the environment.
Regulatory standards for radiological stressors
For planned exposure situations, which is the case for the environmental INPRO assessments, exposures and risks are subject to control to ensure that the specified dose limits for occupational exposure and those for public exposure are not exceeded, and optimization is applied to attain the desired level of protection and safety. Paragraph 1.22 of GSR Part 3 [2] recommends that dose constraints are to be used “for optimization of protection and safety, the intended outcome of which is that all exposures are controlled to levels that are as low as reasonably achievable, economic, societal and environmental factors being taken into account”. It is further clarified that [2]:
“Dose constraints are set separately for each source under control and they serve as boundary conditions in
defining the range of options for the purposes of optimization of protection and safety. Dose constraints are not dose limits: exceeding a dose constraint does not represent non-compliance with regulatory requirements,
but it could result in follow-up actions.”
Although the dose limits, expressed as an annual effective dose to the representative person, are generally
set at the level of 1 mSv, dose constraints are quite different in different countries (see Table 12, in Section II.2.3).
It should be emphasized that there are no constraints for the release of individual radionuclides. There is
also no international recommendation on the limits for concentrations of radionuclides in the environment. On the
other hand, there are so called reference (or authorized discharge limits [22]) levels for radionuclide discharges into
the environment. These reference levels are facility and site specific, and are the result of environmental analyses
with the objective to identify, with sufficient conservatism, allowable emission rates of radionuclides, so as to not
exceed dose limits.
Primarily, the effective dose to human population/non-human species can be considered as an indicator
of environmental impact of radiation release. Ambient radionuclide concentrations in terms of both individual
radionuclides and total alpha, beta or gamma activity can also be chosen to characterize radionuclides as a stressor
specific to nuclear power (see Table 2).
During normal operation of NES facilities, only small amounts of radioactive materials are released into the
environment, resulting in minor radiological impacts on the general public and the environment, i.e. the regulatory
limits are met with high margins [5].
Stressors | Parameters characterizing stressors | Units | Models |
---|---|---|---|
Radionuclides | Total alpha/beta activity in the environments of interest | Bq/m3 | Ref. [23]/National models |
Radionuclide activity concentrations in some appropriate media | Bq/m3 | Ref. [23]/National models | |
Annual effective dose to the population | mSv/a | Ref. [23]/National models | |
Doses to reference biota species | mGy/a | ERICA, RESRAD-Biota models [24] | |
Toxic chemicals | Heavy metals | kg/m3 | National models |
Organic compounds | kg/m3 | National models | |
Land commitment | Land temporally committed | m2/tU (or GW(e)) | None |
Land permanently committed | m2/tU (or GW(e)) | None | |
Particulates | Concentration of particulates released by facility into the air | g/m3 | National models |
Heat | Heat rejected by a facility per year | MW(th)/a | National models |
Solids | Concentration of solids dissolved in effluents | g/m3 | National models |
Concentration solids suspended in water | g/m3 | National models |
Regulatory standards for chemical stressors
In most countries, there are no regulatory limits (standards) for the emission of toxic chemicals into the environment. On the other hand, in most countries there are limits of toxic substances related to their ambient concentrations. Based on such considerations, environmental authorities of some countries establish reference levels for the emission rates of different chemicals, which are evaluated based on analysis of pollutant dispersion using environment models (computer codes).
Examples of stressors
Table 2 presents the NES stressors, the parameters characterizing the stressors that are limited by regulatory requirements and the available analytical models to calculate the impact of stressors.
Necessary INPUT for an INPRO assessment in the area of environmental impact of stressors
This section defines the necessary input and its sources for assessment of an NES in the INPRO methodology area of environmental impact of stressors.
Specification of the nuclear energy system
A prerequisite for INPRO assessment is the specification of the NES (see the introductory manual of the
updated INPRO methodology) to be assessed. The NES is to be defined by the INPRO assessor.
For an environmental assessment, in principle, the following aspects should be covered in the specification
of the NES:
(a) The complete NES should be considered, covering the entire front end of the fuel cycle, the energy conversion unit (reactor) and the entire back end. For all nuclear facilities, the complete lifetime should be covered, i.e. construction, operation and decommissioning.
(b) A global approach should be used, i.e. no geographical boundaries should be introduced that limit the environmental assessment.
(c) If necessary, environmental burdens may be divided into national (or regional) and those occurring outside the country (or regional) borders if such information would be required by stakeholders.
Thus, by meeting the above defined requirements, the general underlying principle of assessing the entirety
of environmental impacts would not be violated.
However, in general, cut-offs, i.e. selection of specific facilities of an NES, will be required in an INPRO
assessment. Such an approach is recommended for nuclear technology users and specifically in the case when a
State is embarking on a nuclear power programme, i.e. in such a country that the INPRO assessment in the area of
environment may consider only the first nuclear power plant and related waste management facilities, assuming
that in the later stages of the nuclear power programme, when more experience is accrued in the country, the scope
of further INPRO assessments will be expanded.
A nuclear technology developer may focus the environmental assessment (analysis) on the design of the
nuclear facility under development.
Information on environmental stressors
An INPRO assessor should have access to relevant design information, i.e. a list of all stressors together with
their levels (e.g. emission rates of radionuclides and chemical toxins, size of land use, etc.), of all NES facilities
to be assessed. This information should be available in design reports. The assessor should further have access
to information on the environmental characteristics of the sites planned for the NES facilities to be assessed.
This information should be available from the responsible government organization or utility.
An INPRO assessor should also have access to the same relevant design information of facilities of an
existing NES that are comparable to the NES facilities to be assessed. Such facilities are called comparable current
facilities in this publication. A comparable current facility means an operating facility of a given type which is
located on a site with environmental characteristics that are comparable to the NES facility assessed and licensed
according to the requirements comparable to the NES facility assessed. For such a comparable current facility,
a complete list of all its stressors and their licensed levels, e.g. emission rates of radionuclides and toxic chemicals,
should be available to the INPRO assessor from the potential supplier. The assessor should also have access to
important environmental characteristics, e.g. wind pattern, population density, etc., and the regulatory requirements
applied for such current facilities. The assessor should further have access to and knowledge of the environmental
standards of the country (or region) where the NES is (planned) to be installed. The national standards should be
available from the responsible government organization (e.g. via its web site). The same information is needed for
the comparable current facilities (if such an approach is planned to be used in the assessment).
The INPRO assessor should also have information to compare the radiotoxicity of release of all radionuclides
of the NES assessed with a current NES delivering similar energy products. For the purpose of the INPRO
assessment, the total radiotoxicity of an NES is defined through dose conversion factors for collective dose per unit
of release, as presented in Ref. [23].
Finally, the INPRO assessor should also have to consider the evidence, e.g. in the form of a written argument
to be provided by the potential supplier, to verify that an optimization procedure such as ALARP has been
performed during the design of all NES facilities to be assessed.
Other sources of INPUT
The INPRO assessor should contact the government organization responsible for environmental aspects to
receive information on the status of EIA studies related to nuclear facilities to be installed in the country as part
of the planned NES. If such studies already exist, most of the necessary input for an INPRO assessment should be
documented in them.
If a State is embarking on a nuclear power programme and using the IAEA service Integrated Nuclear
Infrastructure Review, applying the IAEA milestones approach of Ref. [25], the INPRO assessor should contact
the nuclear energy programme implementing organization responsible for this activity to exchange information and
assure coordination of this effort with the INPRO assessment.
INPRO basic principle, user requirements and criteria in the area of environmental impact of stressors
This section presents the BP, the URs and the CRs in the INPRO methodology area of environmental impact of stressors.
INPRO basic principle: acceptability of expected adverse environmental effects
INPRO basic principle in the area of environmental impact of stressors: The expected adverse environmental
effects of an NES should be well within the performance envelope of current NESs delivering similar energy
products.
Adverse environmental effects may arise from any facility and life cycle stage of an NES. Moreover, the
design and operation of one facility of an NES can have a major influence on the environmental effects of other
facilities. Therefore, in principle, the environmental performance of a proposed NES should be evaluated as an
integrated whole (see the footnote in Section 3.1 on the scope of the INPRO assessment in the area of environmental
impact of stressors).
The BP expresses an expectation that the environmental performance of the NES assessed will be better
than that of a current NES, which is an existing NES comprising facilities of the latest design that is licensed and
operating at the time that the INPRO assessment is performed. A current NES may or may not comply with the
current standards, depending on whether the current standards are different from those that were applied when the
current NES was implemented. However, as further clarified through the first user requirement, UR1, in Section 4.2,
the BP requiring that adverse environmental effects of an NES “should be well within” the performance envelope
of current NES implies, among others, that the expected adverse environmental effects of an NES assessed should
be within the current regulatory standards. Current standards are those prevailing at the time of the INPRO
assessment, and are normally met by recently licensed facilities. In some circumstances, it may be appropriate to
use an environmental standard that is expected to apply when the NES will be implemented.
Figure 3 provides some clarification of the BP. Each stressor caused either by an NES to be assessed — called
an “innovative nuclear energy system” in the figure — or by a current NES chosen for comparison, is represented
by a vector whose length is proportional to the level of the stressor, e.g. the release rate of a radioactive nuclide.
The radius of the circle passing along the vector represents the environmental standard for that stressor. In this way,
each stressor can be represented relative to its standard, and all standards will lie on the circumference (red circle
in Fig. 3). The number of stressors illustrated is arbitrary, and the relative magnitude of vectors representing
different stressors has no specific meaning.
Stressors arising from the current NES are shown as blue arrows, and their magnitudes (levels) are denoted
by LCNS-i.
The green arrows represent the stressors arising from an (innovative) NES to be assessed, and their magnitudes
(levels) are denoted by LNES-i. The BP assumes that each of the NES environmental stressors must be located inside
the red circle (i.e. must meet its standard) representing the current standards.
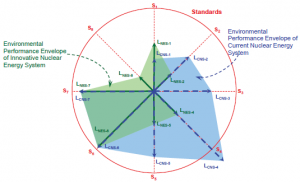
The level of stressors of a current NES may or may not be entirely inside this red circle, depending on
whether the current standards are different from those that were applied when the current NES was implemented,
as illustrated by LCNS-4.
As shown in the figure, some stressors arising from an (innovative) NES to be assessed may have a lower
magnitude (LNES-2, LNES-4, LNES-5) than the current NES, while some of them may be higher (LNES-1) or the same
(LNES-6, LNES-7). In the (innovative) NES to be assessed, some stressors from the current NES may be eliminated
(one is illustrated by LNES-3), while some new stressors (one is illustrated by LNES-8) may occur. Note that neither
the magnitudes of the blue or green areas nor the angles at which the vectors are drawn represent any real quantity,
they are for visualization only.
When all stressors are considered, the performance envelope of an (innovative) NES to be assessed
(green area) should be within the performance envelope of the current NES (blue area).
In case one (or more than one) of the stressors of the (innovative) NES to be assessed compares unfavourably
with the corresponding stressor of the current NES, multivariate analysis might be a possible tool to determine
the degree to which the NES to be assessed is within the current NES environmental performance envelope. An
alternative approach would be to express the level of all stressors commensurately so that they may be accumulated
into a single ‘figure of merit’. Both methods would introduce subjective judgements, but both methods would also
be useful for comparisons of one NES to be assessed to another.
When stressor levels, e.g. dose to the population or ambient concentrations of chemicals, are used as part of a comparison between different NESs, it is important to normalize the stressor levels to per unit energy values. Summarizing the statements above, the BP requires that the spectrum of environmental burdens of an (innovative) NES to be assessed remains within the performance envelope of the current NES (see Fig. 3). Thus, operating technology systems will not be substituted by others that are performing worse, but by technologies that tend to reduce environmental damages instead. The INPRO methodology has defined three user requirements UR1, UR2 and UR3 for the BP.
User requirement UR1: controllability of environmental stressors
User requirement UR1: The environmental stressors from each facility of an NES over the complete life cycle
should be controllable to levels meeting or below current standards.
As stated previously, any energy system will inevitably introduce stressors to the environment, such as
emission of radionuclides or non-radioactive (toxic) chemicals into the air and water, land use and depletion of
non-renewable resources, with potentially adverse environmental effects on a local, regional or even global scale.
UR1 asks that a designer (supplier) of an NES (or a facility thereof) provides controllability of all stressors
throughout the system. Controllability can be achieved by provision of appropriate systems (e.g. filters to reduce
emissions) and monitoring equipment (e.g. instrumentation and control equipment to measure release rates) [26, 27].
The operators of proposed nuclear facilities and processes will be responsible for controlling (and documenting)
the level of stressors during the lifetime of each facility of the NES.
All stressors of an NES facility should be controllable to levels meeting or below current regulatory standards,
i.e. those prevailing at the time the proposed NES is being assessed. In the following, guidance is provided to the
INPRO assessor on how to perform an assessment of CRs related to UR1, assuming the INPRO assessor is a
nuclear technology user planning to install, in their country, nuclear facilities supplied by a nuclear technology
holder.
If an EIA has already been performed for the NES facilities to be assessed and accepted by the authority in
the country as part of the site licensing process, CRs related to UR1 should be automatically met, i.e. no further
application of the INPRO methodology should be needed; however, the INPRO assessor should review the
corresponding reports and confirm that the EIA was performed with due consideration of international guidance
documents (e.g. Refs [28, 29]) and agreements (e.g. Refs [11–13]), and document a summary of the results of this
EIA in the assessment report.
As a guiding objective for the protection of the environment, theoretically, consideration should be given
to the widest possible spectrum of stressors. However, for practical reasons, the degree of inclusion of different
stressors in an INPRO assessment has to be limited.
The first step of determining the level of stressors of an NES facility is to produce a list of all relevant
stressors. For facilities of the front and back end stages of current NESs, an IAEA publication issued in 1996
presents an overview of major stressors, e.g. heavy metal intrusion into surface water and groundwater from mill
tailings and conversion sludge, and mitigation thereof by, for example, effluent treatment [30]. More recent results
of the studies, focused on specific details or new technologies, can be found in the proceedings of conferences or
scientific publications (see Refs [31, 32]).
In Appendix I, examples are provided to the INPRO assessor on which stressors to focus, in the form of tables
that list the important stressors of the facilities of an NES consisting of a water cooled reactor using uranium
oxide (UOX) and MOX fuel. Appendix I also presents brief descriptions of the processes in the individual nuclear
fuel cycle facilities.
Three types of stressor are covered by UR1 and defined in the tables of Appendix I, namely:
- Exposure of the public from radionuclides released to air and/or water (CR1.1);
- Radiation exposure of biota species (CR1.2);
- Other stressors such as ambient concentrations of toxic chemicals to air and/or water, land use, waste heat rejected, dust, etc. (CR1.3).
The media, i.e. water and/or air, and soil, that the stressors are impacting on are listed in the tables of
Appendix I.
Use of a simplified environmental analysis
If an EIA has not yet been performed for the NES facilities to be assessed, it has not yet been accepted
by the regulatory authority or the radiation exposure data of the facility assessed are not available, the INPRO
assessor is offered an option to continue the environmental evaluation of the NES by trying to perform a simplified
(conservative) environmental analysis, as presented in Appendix II, to confirm whether the NES facilities to be
assessed will meet national regulatory standards at their planned site.
Use of a comparable current facility
In some particular situations, the simplified environmental analysis may be avoided for the purpose of INPRO
assessment, even if the EIA has not yet been completed, assuming that the INPRO assessor considers an NES
facility to be based on proven technology. Proven nuclear technology requires that a reference facility — called a
comparable current facility — exists that is licensed based on an EIA and that is in operation. The environmental
behaviour of such a comparable current facility in comparison to the NES facility to be assessed can be used in the
INPRO assessment.
UR1 is satisfied if, in all NES facilities, the levels of all important stressors (listed in Appendix I) are equal
or lower than those of comparable current facilities and, additionally, the NES facility satisfies all of the following
conditions:
- In the NES facilities assessed, no new stressors should appear.
- The environmental characteristics of the NES facility sites should be comparable to the ones of current comparable facilities.
- Comparable regulatory standards should have been (or will be) applied to both the current facilities and the NES facilities to be assessed.
To confirm the existence of a current comparable facility, the INPRO assessor should compare the
environmental characteristics of the planned site of the NES facilities with a site of current facilities. Important
environmental characteristics include, for emissions, the stack height of the plant, the hydrological conditions
(e.g. release into a river, a lake or the ocean), geology (type of soil, aquifers, etc.), meteorology (e.g. wind patterns),
human population distribution around the plant, food chains, endangered species, etc. In principle, all (potential)
sites of nuclear installations have different environmental characteristics. However, if important environmental
characteristics of different sites are comparable, the levels of stressors of facilities located at different sites can be
compared, too. A judgement on the comparability of two sites of nuclear facilities has to be performed by an expert
in the area of EIAs.
The INPRO assessor should perform (with support from an expert in environmental analyses) a comparison
of the regulatory requirements used for licensing of the current facilities and the ones applicable for the NES
facilities to be installed in the country.
If these additional conditions regarding new stressors, comparable environmental characteristics and
regulatory standards are not met, then the proposed approach of using the comparison against comparable current
facility is not applicable.
UR1 explicitly requires to be applied to each facility of the proposed NES, over its complete life cycle (with the exception of potential accidents and their consequences). The INPRO methodology has defined three CRs for UR1, as presented in Table 1.
Criterion CR1.1: Radiation exposure of the public
ᅠIndicator IN1.1: Dose to the publicᅠ
|
---|
Dose to a representative person is considered as IN1.1. Depending on the status of the assessed NES deployment, this information may be directly available from the design or licensing documents of the NES to be assessed. If these data cannot be found, a simplified environmental analysis (see Section II.2) should be performed by the assessor. Alternatively, a comparison with a current comparable facility (when applicable) can be used, as described earlier. |
ᅠAcceptance limit AL1.1 for dose to the publicᅠ
|
---|
Based on GSR Part 3 [2], regulatory authorities should define dose constraints as outlined in detail in IAEA
Safety Standards Series No. WS-G-2.3, Regulatory Control of Radioactive Discharges to the Environment [33].
A dose constraint should be set up by the regulatory authority for a single facility on a given site, taking into
account local conditions that are relevant for radiological impact on humans. More details on dose constraints are
given in Section II.2.3.
Use of a current comparable facility |
Criterion CR1.2: Radiation exposure of non-human species
ᅠIndicator IN1.2: Doses to the reference biota speciesᅠ
|
---|
Current international trends in radiation protection show an increasing awareness of the vulnerability of
the environment. These trends also indicate the need to be able to demonstrate (rather than to assume) that the
environment is protected against the effects of industrial pollutants, including radionuclides, in a wider range of
environmental situations, irrespective of any human connection. The system of protection and safety required by
GSR Part 3 [2] provides a basis for protection from the harmful effects of radiation of both the public and the
environment. This procedure is usually accomplished by means of an environmental assessment that identifies the
target(s), defines the appropriate criteria for protection and assesses the impacts on biota. |
ᅠAcceptance limit AL1.2 for doses to the reference biota speciesᅠ
|
---|
In the scientific annexes to the 1996 and 2008 United Nations Scientific Committee on the Effects of Atomic
Radiation (UNSCEAR) reports [38, 39], published data on the exposures and effects on non-human biota have
been evaluated. It has been found that “chronic dose rates of less than 100 μGy·h−1 (2.4 mGy·d−1) to the most
highly exposed individuals would be unlikely to have significant effects on most terrestrial communities” and “that
maximum dose rates of 400 μGy·h−1 (10 mGy·d−1) to a small proportion of the individuals in aquatic populations
of organisms would not have any detrimental effects at the population level”. These values are in agreement with
those reported elsewhere [40, 41], and reflect an international consensus on doses which could be considered as
acceptable for biota species. |
Criterion CR1.3: Impacts of chemicals and other non-radiation environmental stressors
ᅠIndicator IN1.3: Levels of chemicals and other stressorsᅠ
|
---|
Radiological impacts in a particular environment constitute only one type of impact, and, in most cases,
may not be the dominant impact of a particular facility or activity. Furthermore, the assessment of impacts on the
environment needs to be viewed in an integrated manner with other features of the protection and safety system
in order to establish the requirements applicable to a particular source. As there are complex interrelations, the
approach to the protection of people and the environment is not limited to the prevention of radiological effects on
humans and on other species. An integrated perspective has to be adopted to ensure the sustainability, now and in
the future, of agriculture, forestry, fisheries and tourism, and of the use of natural resources. |
ᅠAcceptance limit AL1.3 for levels of chemicals and other stressorsᅠ
|
---|
For toxic chemicals, the national licensing requirements should be used as AL1.3. For the other stressors
listed in Appendix I, national licensing requirements, e.g. the (normalized) amount of heat rejected to the ultimate
heat sink such as a river or ocean, should be used as AL1.3. |
Final assessment of UR1: Controllability of environmental stressors
The tables in Appendix I can be used by the INPRO assessor to check whether all important stressors of NES
facilities have been considered by the designer.
The acceptance limits AL1.1–AL1.3 of CR1.1–CR1.3 are met if, in all NES facilities, the levels of all
important stressors (listed in Appendix I) are in compliance with existing national or international standards.
User requirement UR2: reduction of total environmental impact of emitted radioactivity
User requirement UR2: Total radiotoxicity of radionuclides discharged by the NES assessed should be lower than
that of a current NES delivering similar energy products.
Radiation tends to be considered as a stressor that is specific to nuclear power, and the concerns of the
ecological safety of the nuclear industry are frequently related to the release of radionuclides into the environment.
As different radionuclides released by nuclear facilities, including waste management facilities, differ in terms
of producing detrimental health or environmental effects, the INPRO methodology suggests using the concept of
radiotoxicity to balance the effects of different radionuclides and to combine them in a single integral parameter.
In its simplest interpretation, radiotoxicity can be defined as the ability of incorporated radionuclides to cause
harmful health effects [42]. This simple definition gives a clear understanding of the basic approach; however, it
cannot be directly applied to the emitted radioactivity, as it does not consider the transport of radionuclides between
the emitter and receptors.
In order to take into account environmental pathways, the INPRO approach is based on application of
dose conversion factors for collective dose per unit activity discharged. The approach considers the discharge of
radionuclides into the atmosphere, freshwater bodies and marine water, thus covering some differences in locations
of nuclear facilities. Hence, radiotoxicity is defined in this publication as an activity of a radionuclide in a release
multiplied by a parameter reflecting a measure of hazard from a given radionuclide.
Although the dose conversion factors (or collective effective dose commitments per unit activity discharged)
are lump parameters, they are based on comprehensive calculations where many environmental transfer parameters
and reference input data for such assessments are included. In particular, the data provided in Ref. [23] are given
for both a simple generic model, which can be used to estimate collective doses for the transfer of radionuclides in
the environment originally developed by UNSCEAR [43], and more complex models, which consider some site
specific factors. To provide comparable bases for assessment of new NESs, generic global parameter values are
used with these models.
For atmospheric releases, three exposure pathways are considered: inhalation, ingestion of terrestrial foods
and external radiation from deposited material. A population density is required in this calculation of collective
dose; for the purposes of calculating screening values, a population density of 35 people per km2 is used [23]. This
represents a global average; considerably higher densities can be found in some countries, while lower densities
can be found in others. The foods considered are: grains, green vegetables and fruit, root vegetables, milk and
meat. Global average yields of particular foods are also required and are taken from the compilation of the Food
and Agriculture Organization of the United Nations (FAO). These are the collective effective dose commitment
values calculated using the effective dose coefficients given in Ref. [23]. In most cases, the values are of the same
order as, or in the range of, the collective doses obtained from the more complex models, and are also presented in
annex VII of Ref. [23].
For releases of radionuclides in liquid form, their dispersion and subsequent transfer to humans will vary
considerably depending on the characteristics of the receiving water body. Although, the general model is used,
account is also taken of the transfer of radionuclides to sediments through the use of the sediment distribution
coefficient Kd. The exposure pathways considered are the consumption of drinking water and aquatic foods.
UR2 explicitly requires to be applied to each facility of the proposed NES, over its complete life cycle, and to
consider the total environmental impact from discharged radioactivity.
The INPRO methodology has defined one CR for UR2, which is presented in Table 1.
Criterion CR2.1: Reduction of environmental impact of radiation
ᅠIndicator IN2.1: Total radiotoxicity of radionuclides emitted to the environment from the nuclear energy system assessedᅠ
|
---|
For the purposes of the INPRO assessments, the radiotoxicity Rki,j is defined as the integral activity (Ai,j, in units
of Bq) of radionuclide (i) discharged per GW∙a for each type of release (j) from a given facility (k) multiplied by
the corresponding dose conversion factor for collective dose (DCFi,j , in man Sv/Bq), as given in Ref. [23]: It must be realized that the concept and product of the radiotoxicity assessment used in UR2 (CR2.1) are
different from those required in UR1 (CR1.1). Annual effective dose is used for assessment of the compliance with
radiation safety standards for the public exposure (UR1). Dose conversion factors for the collective effective dose
commitment used for assessments of radiotoxicity are integrated to infinity, providing an IN of the total impact of
the discharge of radionuclides to the environment within UR2. where Nj is the number of radionuclides released to environment j. Then, the total radiotoxicity of the release from the proposed NES (RT) can be calculated by: where M is the number of nuclear facilities that constitute the NES. If only one new facility (e.g. a new nuclear
power plant) is added to an existing NES, the comparison of radiotoxicity should be done only between newly
introduced and former elements of the NESs, as the radiotoxicity of the remainder of the NES remains the same. |
ᅠAcceptance limit AL2.1 for total radiotoxicity of radionuclides emitted to the environment from the nuclear energy system assessedᅠ
|
---|
Total radiotoxicity is an integral parameter, and should be calculated based on the releases of all facilities
constituting the NES, i.e. environmental analysis should be performed at the whole NES level. The acceptance
limit AL2.1 of CR2.1 is met if the total radiotoxicity evaluated for the proposed NES is lower than that of any
current NES which provides the same energy products. |
User requirement UR3: optimization of the measures to reduce environmental impact
After confirming that UR1 and UR2 are fulfilled, the next step of an INPRO assessment is to check whether
the NES facility design has been adjusted by the designer (supplier) to provide optimized protection of the
environment.
GSR Part 3 [2] is based on the ten fundamental safety principles stated in IAEA Safety Standards Series
No. SF-1, Fundamental Safety Principles [44]. Fundamental Safety Principle No. 5 of SF-1 [44] requires
optimization of protection and states that “Protection must be optimized to provide the highest level of safety that
can reasonably be achieved.” More specifically, para. 3.24 of GSR Part 3 [2] requires:
“For occupational exposure and public exposure*, registrants and licensees shall ensure that all relevant
factors are taken into account in a coherent way in the optimization of protection and safety to contribute to achieving the following objectives:
(a) To determine measures for protection and safety that are optimized for the prevailing circumstances, with account taken of the available options for protection and safety as well as the nature, likelihood and magnitude of exposures;
(b) To establish criteria, on the basis of the results of the optimization, for the restriction of the likelihood and magnitudes of exposures by means of measures for preventing accidents and for mitigating the consequences of those that do occur.”
“* Requirements for the optimization of medical exposure are specified in paras 3.162–3.177.”
User requirement UR3: The measures applied to reduce adverse environmental impact attributable to an NES
should be optimized.
It is suggested that the principle of pollution prevention [45] should be applied for this purpose by the
designer, i.e. reduction of the amount and level of the stressor produced in the NES is the most favoured means of
reducing potential environmental impacts.
As stated in Section 4.1, the NES to be assessed should be held to higher environmental standards than a current NES. Therefore, UR3:
- Applies the philosophy of achieving the best environmental performance reasonably practicable for an NES facility to be assessed;
- Covers all adverse environmental effects, not only the radiological effects on humans;
- Continues to recognize that costs incurred to enhance environmental performance should not be greatly disproportionate to the achieved benefit.
The INPRO methodology has defined one CR for UR3, which is presented in Table 1.
Criterion CR3.1: Optimization of the measures to reduce environmental impact
ᅠIndicator IN3.1: Measures to reduce environmental impact of the nuclear energy systemᅠ
|
---|
There are several options available to the INPRO assessor on how to confirm whether the measures to decrease
the environmental impact of stressors of an NES facility are optimized. This INPRO Manual is not intended
to provide any specific recommendations or indicate preferences on which optimization concept and approach
should be used for such a purpose, and relies instead on regional or national expertise in this area. Possible options
demonstrating optimization of the measures decreasing environmental impact are the BATs, the BEP or the best
available technology not entailing excessive costs (BATNEEC) concepts. Other techniques such as the ALARA or
ALARP concepts can also be used by the designer of an NES facility to demonstrate that measures to provide a
reduction of the environmental impacts are optimized depending on the requirements of national regulations and
experience.
The relationship and differences between the BEP and BATNEEC concepts can be summarized as
follows [47]. BATNEEC is construed to mean the provision and proper maintenance, operation, use and supervision
of facilities which are the most suitable for the purposes. The manner in which this is to be achieved is wide
ranging, but with the overall objective that BATNEEC will be used to limit, abate or reduce an emission from an
activity. The focus is on defined activities, though also addressing areas such as treatment of waste. BEP is more
comprehensive, addressing the entire product life cycle through a combination of practices. These practices may
involve producers, importers, distributors, commercial users and the general public, as well as those engaged in
the collection, recovery or disposal of the substance when it enters the waste stream [47]. Detailed introductory
information on the BAT and BEP methods and an example of application are provided in Section III.2. |
ᅠAcceptance limit AL3.1 for optimization of the measures to reduce environmental impactᅠ
|
---|
The acceptance limit AL3.1 of CR3.1 is met if evidence is available to the INPRO assessor that at least one of
the possible optimization approaches was duly applied by the designer of the NES facility assessed. |
Appendix I
Important stressors in nuclear energy systems
Introduction
This appendix, including the tables, is based on Ref. [30]. It presents illustrative lists of important stressors
for facilities of an NES with a water cooled reactor using UOX or MOX fuel.
Important radionuclides, chemicals and other stressors which are relevant for various facilities of an NES
with a water cooled reactor using UOX or MOX fuel are presented in Sections I.2–I.9, based on current experience.
However, it is recommended that, especially, in the case of an innovative design of an NES facility, this list should
be revised based on the actual radionuclide and chemical inventories in the innovative NES facility, their toxicity
and their environmental mobility.
In the case where calculation of the impact of stressors is necessary, it is desirable to maintain a conservative
approach, and to use internationally accepted models, such as those presented in Appendix II, or available licensed
national models. Model descriptions with appropriate references should be documented in the INPRO assessment
report.
The main facilities of an NES with a water cooled reactor using UOX and MOX fuel are as follows: mining
and milling of uranium ore, refining and conversion of uranium ore, enrichment of uranium, fabrication of nuclear
fuel, power generation, reprocessing of nuclear fuel, and radioactive waste storage and disposal including waste
from decommissioning. Some facilities of an NES have greater environmental impacts compared to others; all
facilities have specific stressors and impacts, and therefore each facility should be considered separately. Depending
on the scope of a specific Nuclear Energy System Assessment, only some of the NES facilities could be considered.
It should be mentioned that there are about 200 radionuclides which are specific to the different stages
of the nuclear fuel cycle and the reactor operation and which can cause radiation impacts on the environment.
In the following sections, only relatively long lived radionuclides are mentioned for each facility. However, it
should be recognized that short lived progenies, which are in equilibrium with some parent radionuclides, can
essentially contribute to the doses to public and biota species for many contamination scenarios. Some of these
short lived radionuclides are not discussed here explicitly; however, it is implied that their contributions to doses
have to be taken into account together with the impacts from their parent radionuclides in radiation impact analyses
(e.g. see Appendix II).
Mining of uranium ore
The environmental impacts of mining of uranium ores may involve the disruption of land surface and
water bodies, the release of radionuclides to air and water, the release of heavy metals and acids, the emission of
particulates, etc. In 2011, for example, conventional uranium mining produced 47% of the world’s uranium —
18% from open pits and 29% from underground mines [55]. Non-conventional methods have also been used
for uranium production, such as in situ leaching (ISL), which produced 42% of the world’s uranium in 2011.
The environmental impacts of a uranium mine are potentially numerous and diverse, depending on the mining
technologies used [56].
The environmental impacts of mining activities are measured using several criteria14. Individual dose is
normally used as the criterion for the radiological impact on humans and non-human species. Several other criteria
are used for the non-radiological environmental impact and for the radiological impact on the environment other
than humans.
Usually, baseline studies are conducted before any mining activities are undertaken; these studies are to be
used when establishing environmental quality objectives needed for the construction, operation, decommissioning
and postdecommissioning phases. In other cases, there are pre-established air, sediment, water and groundwater
quality objectives that need to be met. Social, aesthetic, economic and recreational values are met by establishing
requirements to preserve parts of the affected ecosystem, e.g. fish spawning areas and the habitats of endangered
species may need to be preserved for economic or social and ecological reasons.
Radionuclides of concern
Uranium ore contains natural occurring radioactive material (NORM), which includes uranium isotopes (mainly 238U) and uranium progenies (see Fig. 4) such as 230Th and 226Ra. The radioactive decay of the latter nuclides produces 222Rn (an inert gas), which is normally responsible for the biggest part of exposure to workers in the mines and often to the public.
The important radionuclides which have to be considered in the environmental assessment are: 210Pb, 210Po,
226Ra, 222Rn, 228Th, 230Th, 232Th, 234Th, 234U, 235U and 238U. The environmental impact from 227Ac (a decay product
of 235U) is also recommended to be studied. As mentioned above, short lived progenies such as 214Bi and 214Pb
(both progenies of 222Rn, see Fig. 4) are responsible for a large portion of the radiation impact, but are not listed
here because they are always in equilibrium with 222Rn.
For the majority of these radionuclides (except for 222Rn), contamination of water with radionuclides is the
most important exposure pathway. The radioactivity of water is generally stipulated by the dissolved uranium,
thorium, radium and lead ions. However, an aerial pathway can be also important for several radionuclides,
e.g. 226Ra, 230Th and 238U.
Some natural radionuclides, e.g. uranium, provide both radiation and chemical environmental impacts, which
also have to be taken into account in the assessment.
Chemicals of concern
Water contaminated with chemicals (including NORM) is produced by dewatering of underground and open pit mines, surface water runoff from and seepage through the waste rock piles and ore stockpiles, and ISL restoration activities. In situations when the ore includes pyrites, the generation of acid requires neutralization as part of the water treatment process, if this water is planned to be discharged to the environment. Acid generation is a concern of all types of mining because the acid solubilizes and increases the mobility of heavy metals, and for uranium mining, it also mobilizes radionuclides. Utilization of explosives in mining may add some nitrites, nitrates and ammonia to the mine wastewater.
Other stressors
Examples of other stressors are:
- Temporarily committed land, e.g. for a current NES (using an open fuel cycle with light water reactors (LWRs)), this value is estimated to be about 25 ha/GW(e) for open pit mining [30];
- Permanently committed land;
- Dust, originating at exposed ore stockpiles, ore haul roads, etc.
Illustrative list of stressors
An illustrative list of stressors for evaluation of the environmental impact from uranium ore mining is presented in Table 3.
Stressor | Media type | Normalized release units | Average release units |
---|---|---|---|
Radionuclides | |||
Pb-210 | Water* | MBq/tU or MBq/GW·a | MBq/a or MBq/d |
Po-210 | Water | ||
Ra-226 | Air, water | ||
Ra-228 | Air, water | ||
Th-228 | Air, water | ||
Th-230 | Air, water | ||
Th-232 | Air, water | ||
Th-234 | Air, water | ||
U-234 | Air, water | ||
U-235 | Air, water | ||
U-238 | Air, water | ||
Rn-222 | Air | ||
Ac-227 | Air, water | ||
Chemicals | |||
As | Water | kg/tU or kg/GW·a | kg/a or kg/d |
Se | Water | ||
Ni | Water | ||
Nirites | Water | ||
Nitrates | Water | ||
Ammonia | Water | ||
Sulphates | Water | ||
Others | |||
Land temporarily committed | Soil | m2/tU or m2/GW | m2 (total area) |
Land permanently committed | Soil | ||
Dust | Air | kg/tU | kg/a |
Solids dissolved | Water | kg/tU | kg/a |
Solids suspended | Water | kg/tU | kg/a |
* Water comprises surface water as well as groundwater
Milling of uranium ore
Usually, uranium ore is processed close to the mine to limit transportation costs. The typical process for the
extraction of uranium consists of crushing and grinding of the ore, followed by chemical leaching with sulphuric
acid or an alkali carbonate solution. Acid leaching is the more common method; however, some mills use alkaline
leaching when the ore body contains limestone or similar basic constituents, which would consume uneconomic
quantities of acid. The uranium solution is purified and concentrated by ion exchange and/or solvent extraction
technology. The uranium is then precipitated from solution, filtered and dried to produce a concentrate, known as
yellowcake, which contains between 60% and 90% uranium by weight.
Potential sources of impacts on the environment from mill tailings comprise the following types of release:
(i) escape of gaseous radon; (ii) transport of radioactive particulates by the wind; (iii) contamination of surface
water and groundwater through runoff or seepage of radionuclides, heavy metals or other toxic materials; and
(iv) dispersion of tailings over a wide area caused by erosion or flooding.
Radionuclides of concern
At the stage of milling, radionuclides of concern are similar to those which are considered for mining. The tailing slurry is the most significant source of radionuclides. Mill sites in dry areas give rise to effectively no liquid effluents. However, the runoff water from mills in wet climates will contain radionuclides and may need treatment before release into watercourses. The tailings are characterized by their relatively large volumes and relatively low activity concentrations of long lived natural occurring radionuclides. About 15% of the total radioactivity which was originally contained in the ore is retained in the yellowcake produced by the mill. Once shorter lived radioactive nuclides have decayed, some 70% of the radioactivity originally present in the ore is left in the tailings. The tailings contain nearly all of the naturally occurring radioactive progenies from the decay of uranium, notably 226Ra and 230Th. The presence of 230Th provides a long term source of radon emission. Radioactive airborne effluents from milling may include dusts and radon gas released into the air from ore stockpiles, crushing and grinding of ore, drying and packing of yellowcake, and the tailings retention system. The releases of dusts produced in the processing operations are reduced by ventilation extract scrubbers. Tailings may be a continuing source of radon and radioactive dust after milling operations have ceased. Thus, the list of radionuclides that have to be evaluated for milling is similar to the one for mining.
Chemicals of concern
Contaminated water is discharged from uranium mills to tailing management facilities. The contaminants
include heavy metals, sulphates, chlorides, organics and ammonia. The exact mixture will depend on such factors
as type of process used and ore grade, and should be evaluated separately for each case.
Airborne chemical contaminants released to the environment include combustion products (oxides of
carbon, nitrogen and sulphur) from the process steam boilers and power generation, sulphuric acid fumes in small
concentrations from the leach tanks and vaporized organic reagents from the solvent extraction ventilation system.
In addition, from some plants where sulphuric acid is made on-site, sulphur dioxide is released to the atmosphere.
Other stressors
It is estimated that currently an average of about 4 ha of land area is required for milling of uranium ore sufficient to produce 1 GW(e) of energy in a once through fuel cycle. About 75% of this land is devoted to impoundment for the permanent disposal of mill tailings. The amount of land required is highly dependent on the grade of ore being processed. Lower grade ore milling operations normally use more land per GW(e) compared with facilities processing higher grade ore.
Illustrative list of stressors
An illustrative list of stressors for evaluation of the environmental impact from uranium ore milling is presented in Table 4.
Stressor | Media type | Normalized release units | Average release units |
---|---|---|---|
Radionuclides | |||
Pb-210 | Water | MBq/tU or MBq/GW·a | MBq/a or MBq/d |
Po-210 | Water | ||
Ra-226 | Air, water | ||
Th-228 | Water | ||
Th-230 | Air, water | ||
Th-232 | Water | ||
Th-234 | Water | ||
U-234 | Water | ||
U-235 | Water | ||
U-238 | Water | ||
Rn-222 | Air | ||
Ac-227 | Water | ||
Dust (total alpha activity) | Air | ||
Chemicals | |||
Heavy metals | Water | kg/tU or kg/GW·a | kg/a or kg/d |
Organic | Water | ||
Chlorides | Water | ||
Ammonia | Water | ||
Sulphates | Air, water | ||
Others | |||
Land temporarily committed | Soil | m2/tU | m2 (total area) |
Land permanently committed | Soil | ||
Dust | Air | kg/tU | kg/a |
Solids dissolved | Water | kg/tU | kg/a |
Solids suspended | Water | kg/tU | kg/a |
Refining and conversion of uranium ore to uranium hexafluoride
Uranium ore concentrate (UOC) may consist of any of several different uranium compounds. It still contains elements other than uranium and some uranium radioactive decay products. UOC is refined to obtain higher purity uranium compounds. Two different processes — wet and dry — are used to purify UOC and convert it into a usable form. The more commonly used process, the wet process, consists of dissolving UOC in nitric acid and refining it by solvent extraction. The pure uranyl nitrate formed is then converted to uranium trioxide, which can be converted to uranium dioxide (UO2) for natural uranium fuel, or converted progressively to uranium tetrafluoride and uranium hexafluoride (UF6) for enrichment to produce LWR fuel
Radionuclides of concern
Emissions from uranium refining and conversion facilities are relatively minor. The only radioactive emission
of significance is natural uranium, which may be emitted as dust or as a volatile reaction product of UF6 [30]. The
plants are equipped with dust collectors and scrubbers to mitigate the impacts of these emissions.
The effluents from the wet and dry processes of UF6 production differ substantially. In the wet process, most
of the impurities entering with UOC are rejected in the raffinate solution from solvent extraction, whereas in the
dry process, most of the UOC impurities are contained in solid wastes from the fluorination and distillation stages.
In the case when raffinate from the wet process is recycled through the uranium mill where residual uranium
is recovered and the remaining waste is disposed of with the tailings, the radiological impact of the raffinate is
included in the radiological impact of the milling facility. Where the raffinate is not recycled, the radiological
impact is due to residual thorium and, to a lesser extent, radium.
Chemicals of concern
Atmospheric emissions from the refining and conversion processes contain greenhouse gases such as NOX. Aqueous release may contain residual tributylphosphate and heavy metals that could have a potential impact on benthic fauna. Other contaminants with potential for environmental impacts are nitrates, and, under accident conditions, ammonia and fluorides may also be released.
Other stressors
Of the temporary land commitment for a UF6 production plant (~1.3 ha/GW(e)), approximately 10% is needed for roads, fills and plant structures [30]. Approximately 1% of land needed for a plant is permanently committed for waste burial.
Illustrative list of stressors
An illustrative list of stressors for evaluation of the environmental impact from refining and conversion is presented in Table 5.
Stressor | Media type | Normalized release units | Average release units |
---|---|---|---|
Radionuclides | |||
Pb-210 | Water | MBq/tU or MBq/GW·a | MBq/a or MBq/d |
Po-210 | Water | ||
Ra-226 | Air, water | ||
Th-228 | Air, water | ||
Th-230 | Air | ||
Th-232 | Air | ||
Th-234 | Air, water | ||
U-234 | Water | ||
U-235 | Air, water | ||
U-238 | Air, water | ||
Chemicals | |||
Heavy metals (have to be specified within the assessment) | Water | kg/tU or kg/GW·a | kg/a or kg/d |
Fluorides | Air, water | ||
Nitrogen oxides | Air | ||
Sulphur oxides | Air | ||
Carbon monoxide | Air | ||
Volatile organic compounds | Air | ||
Tributylphosphate | Water | ||
Others | |||
Land temporarily committed | Soil | m2/tU or m2/GW | m2 (total area) |
Land permanently committed | Soil | ||
Heat rejected | Air | MW(th)/tU | MW(th)/a |
Solids dissolved | Water | kg/tU | kg/a |
Solids suspended | Water | kg/tU | kg/a |
Enrichment of uranium
LWRs normally use uranium enriched to about 4–5% in 235U. Current commercial enrichment technologies are based on gaseous diffusion or centrifugation of uranium isotopes in the form of UF6. Reference [57] briefly explains the gas diffusion process as follows:
“In the gaseous diffusion process, gaseous UF6 is compressed and passed over a porous membrane. Molecules of UF6 containing the lighter isotope, uranium-235, diffuse through the membrane more rapidly than those with the heavier uranium-238 isotope; consequently, the UF6 passing the membrane has a slightly greater proportion of molecules containing uranium-235. The degree of enrichment for one membrane is minute and over a thousand successive diffusion stages are necessary to raise the proportion of uranium-235 from the naturally occurring level of 0.71 per cent to the 2–4 per cent required in the product stream, with about 0.25 per cent in a reject ‘tails’ stream.
“Each stage requires recompression of the gaseous hexafluoride. Uranium enrichment by gaseous diffusion requires large quantities of electrical energy. About 36.5 MWe-y of electricity would be needed by a gaseous diffusion plant to enrich the uranium for the generation of 1 GWe of electricity in a LWR”.
For the the uranium enrichment by centrifugation process, Ref. [57] provides the following simplified description:
“During enrichment by gas centrifugation, molecules of UF6 containing the heavier isotope uranium-238 migrate preferentially to the wall of a rapidly rotating cylinder. There is a consequent enrichment in the lighter uranium-235 isotope in the gas near the tube axis. The separation factor is greater than in the diffusion process, and the two streams removed from the tube axis and wall require only tens of stages arranged in a cascade to produce the required percentages of uranium-235 in the product and the reject tails. High centrifugal stresses limit the size of the equipment, and many parallel cascades involving hundreds of thousands of centrifuges are required to achieve the separative capacity of a commercial enrichment plant.”
Radionuclides of concern
Emissions of radionuclides from the enrichment process are generally small and consist essentially of long lived uranium isotopes, such as 234U, 235U and 238U, together with 234mPa and 230Th, which are the short lived decay products of 238U. The long half-life of 230Th prevents activity buildup of any other radionuclide of the 238U series. For current facilities, the radiological impacts on the public and non-human species are negligible. At the Capenhurst enrichment site in the United Kingdom, doses to the critical group members of the public are estimated to be less than 0.01 mSv per year.
Chemicals of concern
The enrichment plant itself generates small quantities of airborne fluorides and oxides of nitrogen and sulphur from the process cooling systems, process cleanup operations, on-site steam plant and auxiliary production facilities. Uranium losses in the effluents are very low. Some sludge from container cleanup operations is usually retained on-site. The depleted uranium residue from enrichment plants is normally stockpiled for possible future recovery of the remaining fissile material. The depleted uranium can be used in the future as a resource, and is therefore not considered as a release (or waste). It is noted that near current enrichment facilities, the concentrations of gaseous and liquid effluents are below the ranges for which deleterious effects have been observed.
Other stressors
Essentially, none of the land required for enrichment facilities is committed permanently. The temporary land requirement is estimated to be 0.3 ha/GW(e). Water is required for the operation of cooling towers associated with the gaseous diffusion process and also for the large amounts of electricity that need to be generated. For enrichment by gaseous diffusion, 92% of all the electricity required in a nuclear fuel cycle up to fuel fabrication is consumed in enrichment. Other enrichment techniques such as centrifuges require less than one tenth of the electricity needed for a gaseous diffusion plant.
Illustrative list of stressors
An illustrative list of stressors for evaluation of the environmental impact from an enrichment plant is presented in Table 6.
Stressor | Media type | Normalized release units | Average release units |
---|---|---|---|
Radionuclides | |||
Ra-226 | Water | MBq/tU or MBq/GW·a | MBq/a or MBq/d |
Rn-222 | Air | ||
Tc-99 | Air, water | ||
Th-234 | Air | ||
U-234 | Air, water | ||
U-235 | Air, water | ||
U-238 | Air, water | ||
Chemicals | |||
Fluorides | Air, water | kg/tU or kg/GW·a | kg/a or kg/d |
Nitrogen oxides | Air | ||
Sulphur oxides | Air | ||
Carbon monoxide | Air | ||
Volatile organic compounds | Air | ||
Tributylphosphate | Water | ||
Others | |||
Land temporarily committed | Soil | m2/tU or m2/GW | m2 (total area) |
Land permanently committed | Soil | ||
Dust | Air | kg/tU | kg/a |
Dust (radioactive) | Air | MBq/tU | MBq/a |
Solids dissolved | Water | kg/tU | kg/a |
Solids suspended | Water | kg/tU | kg/a |
Conversion to uranium dioxide and fuel fabrication
The required material for the fabrication of fuel for an LWR is UO2. The UF6 (enriched to about 4–5% in 235U) is converted to UO2 powder, which is formed into pellets, sintered to achieve the desired density and ground to the required dimensions. Fuel pellets are loaded into tubes of zirconium alloy (zirconium–tin or zirconium–niobium), which are sealed at both ends. These fuel rods are spaced in fixed parallel arrays to form reactor fuel assemblies.
Radionuclides of concern
The radionuclides of concern are about the same as those described in Section I.5 for enrichment. The most important pathway of the population and non-human species exposure is inhalation. The doses due to liquid discharges are much less than those from airborne discharges.
Chemicals of concern
The effluent from fuel fabrication with the greatest potential environmental impact is chemical in nature.
Hydrogen fluoride (HF) is potentially the most significant airborne chemical effluent from fuel fabrication.
Liquid effluent from fuel manufacture contains nitrogen compounds formed from ammonia in the production of
UO2 powder and by nitric acid in the scrap recovery operations. Very small quantities of uranium are released with
the effluent gases and liquids. Ammonia and nitrates are found in liquids released from the waste holding ponds.
In a dry fuel production process, the emissions of HF to the environment are very small. Nearly all of the
HF produced will be removed from the off-gas by the condensing and cleaning system. The end products, liquid HF
and CaCO3/CaF2, are suitable for industrial use. Thus, the non-radiological impacts are mitigated by the recovery
and recycling of potential contaminants.
Other stressors
The temporary land requirement is estimated to be 0.1 ha/GW(e) for an NES with an LWR reactor. All of the land required for fuel fabrication can be reclaimed by conventional techniques. Care would have to be taken when decommissioning the holding ponds or lagoons. The facility requires water, most of which is used for cooling of plant processes. The water does not come into contact with uranium or process chemicals during operation. In the dry process, there is no cooling water discharge.
Illustrative list of stressors
An illustrative list of stressors for evaluation of the environmental impact from UO2 fuel fabrication is presented in Table 7.
Stressor | Media type | Normalized release units | Average release units |
---|---|---|---|
Radionuclides | |||
Ra-226 | Air | MBq/tU or MBq/GW·a | MBq/a or MBq/d |
Th-234 | Air | ||
U-234 | Air, water | ||
U-235 | Air, water | ||
U-238 | Air, water | ||
Chemicals | |||
Fluorides | Air, water | kg/tU or kg/GW·a | kg/a or kg/d |
Nitrogen oxides | Air | ||
Ammonia | Water | ||
Others | |||
Land temporarily committed | Soil | m2/tU or m2/GW | m2 (total area) |
Land permanently committed | Soil |
Mixed oxide fuel fabrication
Two processes are currently used to produce MOX fuel; these processes differ mainly at the beginning,
owing to the nature of their feed materials. For the dry process, the feed materials are UO2 (ammonium uranyl
carbonate, ammonium diuranate and integrated dry route) and PuO2 powders. The alternative wet process starts
with plutonium and uranium nitrate solutions. MOX powder is prepared by co-milling or co-conversion, depending
on the type of feed material.
Following the main fabrication, the steps (pelletizing, sintering, rod fabrication and assembling) are
comparable with those of uranium fuel fabrication. The main difference to UO2 fuel fabrication is related to a strict
alpha activity containment of the processed material in tight gloveboxes and shielding against gamma and neutron
radiation.
Radionuclides of concern
Atmospheric discharges result from ventilation — for dynamic containment purposes — of production
buildings and gloveboxes wherein the manufacturing process is performed. The discharges consist of aerosols of
uranium/plutonium.
Very low alpha active liquids, principally from cleaning of non-contaminated or very low contaminated areas
(operators, floors, etc.), are discharged into the environment after nuclear control measurements (range of activity:
1–5 kBq/m3). The balance of discharged activity is exactly maintained.
Liquid discharges increase with the throughput of a plant (these discharges mainly depend on the number of
workers and the surface of the buildings); typical discharged activities are in the range 0.5–3 MBq/a.
Chemicals of concern
The chemical emissions from this process are negligible (for the dry process, there are no chemical emissions).
Other stressors
Basically, MOX fuel fabrication is a recycling activity. Plutonium is a valuable fissile material originating from the reprocessing of UO2 spent fuel. The replacing of 235U by plutonium in LWR fuel contributes to a more efficient use of uranium resources. In most cases, for the fuel matrix, natural uranium, depleted uranium and reprocessed uranium can be used. Utilization of other resources (e.g. energy, fluids and land) is comparable with those used in UO2 fuel fabrication. The land requirement is estimated to be 3 ha/GW(e). The dry process does not use water, and its energy requirement is estimated to be 0.3 MW/a per GW(e).
Illustrative list of stressors
An illustrative list of stressors for evaluation of the environmental impact from MOX fuel fabrication is presented in Table 8.
Stressor | Media type | Normalized release units | Average release units |
---|---|---|---|
Radionuclides | |||
Pu | Air | MBq/tU or MBq/GW·a | MBq/a or MBq/d |
U-234 | Air, water | ||
U-235 | Air, water | ||
U-238 | Air, water | ||
Others | |||
Land temporarily committed | Soil | m2/tU or m2/GW | m2 (total area) |
Land permanently committed | Soil |
Power generation
Radionuclides of concern
Discharges from nuclear reactors (LWRs and heavy water reactors) to the atmosphere consist mainly of the
noble (inert) gases: 41Ar, 85Kr, 85mKr, 88Kr, 131Xe, 133Xe, 133mXe, 135Xe and 138Xe. Most of these radionuclides, except
for 85Kr with a half-life of 10.8 years, are short lived, and their environmental impacts are essentially limited. Other
radioactive gases include 14C, 3H and 131I.
Radionuclides in liquid discharges with potential environmental impacts consist of 134Cs, 137Cs, 51Cr, 58Co,
3H, 55Fe, 54Mn, 110mAg and 65Zn.
Examples of the most important radionuclides that can be discharged from nuclear power plants are briefly
discussed in Ref. [29]. A UK study [58] provides detailed information on discharges of radionuclides from two
water cooled reactors of latest design, namely the European pressurized reactor (EPR) (AREVA) and the AP1000
(Westinghouse) reactor. Data on estimation of releases of radionuclides from the AP1000 reactor, the EPR, the
economic simplified boiling water reactor (ESBWR) (GE Hitachi) and the ACR-1000 reactor (Atomic Energy of
Canada Limited), and operational data for their predecessor designs, are summarized in Ref. [59].
Chemicals of concern
Atmospheric emissions of chemicals from this process are negligible. Aqueous releases may contain sewage with organic compounds and heavy metals originating from auxiliary facilities, such as radioactive waste facilities, the condensate polishing plant, the steam generator blowdown system, the laundry, laboratories, etc. The Environment Agency provides comprehensive information on discharges of chemicals from the EPR and the AP1000 reactor in Ref. [60].
Other stressors
Other stressors are: temporary land requirement estimated to be about 50 ha for a 1.2 GW(e) nuclear power plant [61]; use and depletion of water resources and disturbance of the hydrological state of surface water and groundwater; heat discharge; and changes in the biodiversity of non-human species in the terrestrial and aquatic ecosystems in the vicinity of a facility.
Illustrative list of stressors
An illustrative list of stressors for evaluation of the environmental impact from power generation is presented in Table 9.
Stressor | Media type | Normalized release units | Average release units |
---|---|---|---|
Radionuclides | |||
H-3 | Air, water | MBq/tU or MBq/GW·a | MBq/a or MBq/d |
C-14 | Air, water | ||
Na-24 | Water | ||
Ar-41 | Air | ||
Cr-51 | Air, water | ||
Mn-54 | Water | ||
Fe-55 | Water | ||
Co-58 | Air, water | ||
Fe-59 | Water | ||
Co-60 | Air, water | ||
Ni-63 | Water | ||
Zn-65 | Water | ||
Kr-85 | Air | ||
Kr-85m | Air | ||
Kr-87 | Air | ||
Kr-88 | Air | ||
Sr-89 | Air, water | ||
Sr-90 | Air, water | ||
Y-91 | Water | ||
Zr-95 | Air, water | ||
Nb-95 | Air, water | ||
Tc-99m | Water | ||
Ru-103 | Air, water | ||
Ag-110m | Air, water | ||
Te-123 | Water | ||
Sb-124 | Water | ||
Sb-125 | Water | ||
Xe-131m | Air | ||
I-131 | Air | ||
I-133 | Air, water | ||
Xe-133 | Air | ||
Xe-133m | Air | ||
Cs-134 | Air, water | ||
Xe-135 | Air | ||
Xe-135m | Air | ||
Cs-136 | Water | ||
Cs-137 | Air, water | ||
Xe-137 | Air | ||
Xe-138 | Air | ||
Ba-140 | Air, water | ||
La-140 | Water | ||
Ce-144 | Air, water | ||
Pr-144 | Water | ||
Pu-241 | Water | ||
Chemicals | |||
Organic compound | Water | kg/tU or kg/GW·a | kg/a or kg/d |
Heavy metals | Air, water | ||
Other chemicals[60] | Air, water | ||
Others | |||
Land temporarily committed | Soil | m2/tU or m2/GW | m2 (total area) |
Land permanently committed | Soil | ||
Heat rejected | Air, water | MW(th)/tU or MW(th)/GW·a | MW(th)/a |
Water quality solids dissolved | Water | kg/tU or kg/GW·a | kg/a |
Direct damage solids suspended | Water |
Reprocessing
The principal objectives of reprocessing are: (i) to recover uranium and plutonium for reuse as nuclear fuels;
(ii) to separate radioactive and neutron absorbing fission products; and (iii) to convert the radioactive wastes of
spent fuel reprocessing into forms that are suitable for safe storage and disposal. The difference between a once
through fuel cycle of an NES and a reprocessing cycle (also called a partly closed fuel cycle) is that the reprocessing
cycle makes more efficient use of the fuel through extraction of plutonium and recycling of the residual 235U.
The spent fuel is first stored to allow its thermal power and its radioactivity to decrease to the design levels
of the reprocessing plant. Generally, for spent LWR fuels, the total cooling time is at least three years. The fuel
cooling pond water can become slightly contaminated, primarily with 137Cs, 60Co, 54Mn and 90Sr, and needs to
be decontaminated by filtration, to remove particulates, and by ion exchange, to absorb the activity present in a
soluble form.
In the following, the currently used industrial (wet) process for reprocessing of spent uranium fuel (plutonium
uranium redox extraction — PUREX) is described. The spent fuel is mechanically chopped into small pieces,
which are dipped into a dissolver solution. The fuel is leached from the chopped cladding by boiling nitric acid.
After leaching, the fuel hulls (chopped cladding) are washed and transferred to a storage. The liquid in the dissolver
will contain some insoluble material, including fission product compounds from the spent fuel and both coarse
and fine particulates from the cladding material, together with debris from the structural components of the fuel
elements. These insoluble residues are separated by centrifugation or filtration. Both these wastes will need to
be stored prior to disposal. The clear solution is analysed to determine its nuclear fuel content and is eventually
adjusted for uranium–plutonium concentration and acidity prior to extraction.
The liquid from the dissolver, after the removal of solids, is passed to successive extraction systems to
separate the fission product wastes from the uranium and plutonium. The products from the solvent extraction
cycles are uranium and plutonium nitrates, while the bulk of the fission products are removed as high level wastes
for storage prior to conditioning and ultimate disposal. The solvent of the washing process is reused. The two
products of the solvent extraction process are first concentrated by evaporation. The uranyl nitrate solution can then
be converted to UOX form. Subsequently, this product can be sent to another site for conversion to UF6 and used in
a re-enrichment cycle. The plutonium nitrate is converted first to plutonium oxalate by a precipitation process and
subsequently to the oxide by thermal decomposition and calcination.
Radionuclides of concern
Reprocessing is one of the most important sources of radionuclide release to the environment. The radionuclides of particular interest in airborne discharges include 137Cs, 14C, 3H and 129I, and in liquid discharges 137Cs, 14C, 3H, 129I, 106Ru and 90Sr. Additionally, reprocessing of nuclear fuel results in the discharge of actinides such as 241Am, 237Np, 239Pu, 240Pu and 241Pu.
Chemicals of concern
Releases of chemicals from reprocessing facilities are negligible. For example, Ref. [30] gives an estimation of discharge of non-radiological liquid effluent from the Thorp reprocessing plant in the United Kingdom on the level that produces pollutant concentrations that are less than 1% of the relevant environmental quality standards.
Other stressors
The temporary land requirement is estimated to be 1.5 ha/GW(e). About 95% of this land use is a fenced-in buffer zone and is not disturbed. The La Hague (France) reprocessing site is a 300 ha complex and its reprocessing capacity is 1600 t/a. It also has 14 400 t of spent fuel storage pools capacity.
Illustrative list of stressors
An illustrative list of stressors for evaluation of the environmental impact from wet reprocessing of nuclear fuel is presented in Table 10.
Stressor | Media type | Normalized release units | Average release units |
---|---|---|---|
Radionuclides | |||
H-3 | Air, water | MBq/tU or MBq/GW·a | MBq/a or MBq/d |
C-14 | Air, water | ||
Na-24 | Water | ||
P-32 | Air, water | ||
Sc-46 | Air, water | ||
Si-41 | Water | ||
Cr-51 | Water | ||
Fe-55 | Air, water | ||
Co-58 | Air, water | ||
Mn-54, 56, 58 | Air, water | ||
Co-60 | Air, water | ||
Cu-64 | Water | ||
Zn-65 | Air, water | ||
As-76 | Air | ||
Kr-85 | Air | ||
Kr-85m | Air | ||
Kr-87 | Air | ||
Zr-95 | Air, water | ||
Nb-95 | Air, water | ||
Sr-90 | Air, water | ||
Ru-106 | Air, water | ||
Sb-124 | Water | ||
I-129 | Air, water | ||
I-131 | Air, water | ||
Xe-133 | Air | ||
Xe-133m | Air | ||
Xe-135 | Air | ||
Xe-138 | Air | ||
Cs-134 | Air | ||
Cs-137 | Air, water | ||
Ba-140 | Air, water | ||
La-140 | Air, water | ||
Ce-141 | Air, water | ||
Ce-144 | Air, water | ||
Eu-152 | Water | ||
Eu-154 | Water | ||
Pu-239 | Air, water | ||
Pu-240 | Air, water | ||
Pu-241 | Air, water | ||
Np-237 | Air, water | ||
Am-241 | Air, water | ||
Chemicals | |||
Organic compound | Water | kg/tU or kg/GW·a | kg/a or kg/d |
Heavy metals | Air, water | ||
Others | |||
Land temporarily committed | Soil | m2/tU or m2/GW | m2 (total area) |
Land permanently committed | Soil |
Storage of spent nuclear fuel away from the reactor
Compared to other nuclear facilities of an NES, rather minor stressors occur in facilities away from reactors for storage of spent nuclear fuel. No chemicals are expected to be released from such a facility. Spent fuel can be stored in dry casks or in fuel ponds. Environmental stressors from a dry storage and a wet storage essentially differ. A preliminary list of stressors for the evaluation of the environmental impact from a wet storage facility is presented in Table 11.
Stressor | Media type | Normalized release units | Average release units |
---|---|---|---|
Radionuclides | |||
Co-60 | Air | MBq/tU or MBq/GW·a | MBq/a or MBq/d |
Cs-137 | Air | ||
Others | |||
Land temporarily committed | Soil | m2/tU or m2/GW | m2 (total area) |
Land permanently committed | Soil |
Disposal of radioactive waste
Releases from radioactive waste disposal facilities including facilities for direct disposal of spent nuclear fuel are discussed in a separate manual of the INPRO methodology covering the NES sustainability assessment in the area of waste management. In this current INPRO Manual on the environmental impact of stressors, the waste disposal facilities are considered in UR2 along with other NES installations contributing to the total radiotoxicity, and in UR3, which is focused on the optimization of measures to reduce environmental impacts.
Appendix II
Simplified environmental analysis
This appendix presents practical advice on how to perform simplified environmental analysis of radiological
stressors based on IAEA [23] and United States Department of Energy (DOE) [24] methods. It further discusses
briefly the analysis of the consequences of toxic chemical releases and presents the results of comprehensive
environmental studies.
Introduction
The INPRO assessment approach using a comparable current facility which was presented for UR1 is not applicable when:
- New radionuclides, chemicals or other stressors different from current facilities exist in an innovatively
designed facility of the NES assessed;
- The level of a stressor in the NES facility assessed is higher than the value of a comparable current facility;
- Significant differences exist between the environmental characteristics of the site of the current facility and the one proposed for the NES facility;
- The regulatory requirements used for the current facility are significantly less stringent than those in the country of the NES assessed.
The INPRO assessor should use a simplified environmental analysis approach when an EIA is not yet
available for the NES facility under assessment and a comparable current facility cannot be identified.
The regulatory requirements relevant to the environmental impact of stressors usually relate to the potential
impacts of pollutants or radionuclides than to the levels of stressors, i.e. the emission rates. In the case of
radiological stressors, the regulatory standards are defined based on the impact of radiation in terms of exposures.
The determination of the impact of stressors, e.g. doses, is required to compare it to current regulatory standards
(e.g. dose limits).
Environmental standards are defined by Member States. In particular, for radioactive emissions from nuclear
facilities, the regulatory body of a Member State is responsible for identifying the values of the dose constraints
and sets discharge authorizations for nuclear facilities or sites, which are usually given in terms of annual limits of
discharge in licensing and operation permits.
Summarizing the statements above, in such cases where no comparable current facility for an NES facility
to be assessed can be found, the INPRO assessor should demonstrate that ALs for the stressors of the NES facility
(current environmental standards including dose constraints in the country where the facility will be installed) are
met, and the environmental impact of stressors should be estimated.
Simplified methods on how to calculate the environmental impacts (doses) of emitted radionuclides on
humans and non-human biota (plants and animals) are presented in next two sections.
IAEA generic models for radiological impact on humans
A simplified calculation based on the IAEA generic models described in Ref. [23] could be conducted to
demonstrate compliance with dose limits (impacts of radiological stressors). This method, called a tiered approach,
consists of a sequence of several tiers with increasing complexity and detail and is discussed in this section.
Similar simplified approaches have been proposed for calculation of impacts from radionuclides in different
contexts. For example, in the DOE graded approach (DOE-GA) model (see Section II.3.3), the RESRAD family
of codes includes a tool for the calculation of doses to humans, which might be used for comparison with the
calculations performed using the IAEA generic models. Reference examples of the application of these simplified
approaches can be found in Refs [2, 23] for humans and in Refs [24, 63–65] for biota.
With regard to doses to humans caused by the discharge of radionuclides into the environment, the IAEA
has developed generic models for the purpose of screening of discharges [23]. They are designed to determine
the likely magnitude of the radiological impacts through a simplified but conservative method. The use of simple
screening models for dose calculation is usually one of the first steps in licensing a nuclear facility [33].
Originally, these methods were expected to be applied mainly for small scale facilities in order to facilitate
easy calculation at low cost, to determine whether further, more refined and expensive analyses could be avoided.
According to Ref. [23]:
“However, for many larger scale nuclear facilities the assessed doses from the screening models presented in this report are more likely to approach the dose limiting criteria set by the Regulatory Authority (e.g. dose constraint), and users are more likely to need to follow a screening calculation with a more realistic, site specific and detailed assessment.”
The goal of the method presented in Ref. [23] is to preliminary calculate doses to the most exposed individuals
in hypothetical critical groups. To achieve this goal, the last year of discharge (assumed the 30th year of operation
in Ref. [23]) is considered in order to maximize doses; the effective doses from external and internal radiation per
unit of concentration of each radionuclide are then calculated.
The application of the method described in Ref. [23] is relatively straightforward. The basic information
required is:
- Quantities and types of radionuclides discharged;
- Mode of discharge and the discharge points, identifying separately discharges to the atmosphere, surface water or sewerage systems.
To ensure that the analysis is consistent, to the extent possible, with the approach for the biological effects on non-human biota based on the DOE-GA [24] (see Section II.3.3), it was decided to include both steps described in Ref. [23] into the first level of the method, called tier 1.
Generic IAEA model tier 1: No dilution and generic environment
In tier 1, a calculation of effective individual doses (during the final year, which, in Ref. [23], is assumed to be
the 30th year of discharge) from external and internal exposure to the most exposed individuals in a hypothetical
critical group is to be performed. There are two steps (or iterations).
Step 1 of tier 1: Application of the ‘No dilution (no dispersion) model’
The maximum predicted annual average radionuclide concentrations in Bq/m3 at the point of discharge in
either air or water are to be used. Effective doses to a hypothetical critical group can be calculated by multiplying
the concentrations at the point of discharge by dose factors (or dose coefficients) in Sv/a per Bq/m3, which are
different for discharges to air and surface water. These are listed in tables I–I and I–II of Ref. [23], and Excel sheets
with these factors are available from the IAEA. These coefficients were derived using established methodologies,
default parameters and dose coefficients [66, 67].
The most exposed individual is hypothesized to be located at the discharge point. The maximum predicted
annual average radionuclide concentrations in either the air or water at the point of discharge are considered.
No dilution screening factors are used to convert exposures into doses.
The calculated maximum dose is compared with the acceptance limit, which is the dose constraint
(see Section II.2.3) for a specific nuclear facility. If no compliance with the acceptance limit is found, the analysis
should be refined with step 2.
Step 2 of tier 1: Application of the ‘generic environmental model’
The concentrations at the location nearest to the facility at which a member of the public will be likely to
have access, or from which a member of the public may obtain food or water, are needed in order to see whether
they match the generic assumptions. The analyst can easily calculate doses for the generic environmental model
by multiplying release rates with generic dose calculation factors (see tables I–III and I–V of Ref. [23]), which
are given in units of Sv/a per Bq/s for discharges into the atmosphere and surface water, and in Sv/a per Bq/a for
discharges into sewers.
For the background calculations, the simplified methods for estimating radionuclide concentrations in air
are outlined in Ref. [23] and will not be reported here. The average radionuclide concentrations in air, food and
water are combined with the annual rates of intake to obtain an estimate of the total radionuclide intake during
that year. This total intake over the year is then multiplied by appropriate dose coefficients to obtain an estimate of
the maximum effective dose, in one year, from inhalation or ingestion. In a similar manner, the concentrations of
radionuclides in shoreline sediments and surface soils are used with appropriate dose coefficients to estimate the
effective dose received from external irradiation during the final operational year of the facility. The effective dose,
in one year, from immersion in a cloud containing radionuclides may be calculated by multiplying the average
concentration in air by the appropriate external dose coefficients. To obtain the total maximum effective dose in the
final operational year, the effective doses from all radionuclides and exposure pathways are summed.
Dilution and dispersion are somewhat taken into account; generic factors are derived using additional
standardized assumptions about the discharge characteristics and location of a hypothetical critical group.
Similar to the no dilution model, the calculated maximum dose is to be compared to an acceptance limit
to confirm acceptable results. As suggested in section 8 of Ref. [23], for the acceptance limit for the generic
environment model, a more stringent value of dose should be used, i.e. one tenth of the dose constraint
(see Section II.2.3).
If no compliance with the acceptance limit (e.g. one tenth of the dose constraint) is met, Ref. [23] suggests
that the site specific discharge conditions and the actual critical group location be taken into account. Within an
analysis, this step would correspond to tier 2.
Generic IAEA model tier 2: Use of site specific characteristics
Application of tier 2 depends on the maturity level of the NES facility being analysed. If no specific environmental data of a proposed site exist, or if the NES has a too low level of maturity, tier 2 cannot be applied, and the need for RD&D should be identified by the analyst. If the maturity level is medium to high, the analysis could be refined by appropriately adjusting the key parameters to the specific situation (e.g. more specific regional dispersion parameters, local/regional food chains) or by using more refined pathway analysis tools. In the case where the site is known, the analyst could consider actual discharge conditions, take into account the location of the actual critical group and make use of specific parameters. If no compliance is achieved in this step, the analyst should identify and propose appropriate RD&D. Default values used within Ref. [23] can be changed to adapt to the characteristics of the region or site and to suit the needs of the analysis.
Dose constraints for radiation protection of humans
In this section, the INPRO AL for impact of radiological stressors on humans is defined. This AL for radiation
protection should be used in the case when no comparison of facilities is necessary, i.e. when an EIA is available or
a simplified analysis has been applied (as discussed in Section II.1).
Table 12 (taken from WS-G-2.3 [33]) shows values of annual dose constraints for a single source (facility)
used in current practices by different Member States. Based on the consideration that the reported values are within
a relatively narrow range, WS-G-2.3 [33] recommends that the annual dose constraint should not exceed 0.3 mSv
(see also Ref. [68]).
Member state | Dose constraint (mSv/a) | Source |
---|---|---|
Argentina | 0.3 | Nuclear fuel cycle facilities |
Belgium | 0.25 | Nuclear reactors |
China | 0.25 | Nuclear power plants |
Italy | 0.1 | Pressurized water reactors |
Luxembourg | 0.3 | Nuclear fuel cycle facilities |
Netherlands | 0.3 | Nuclear fuel cycle facilities |
Spain | 0.3 | Nuclear fuel cycle facilities |
Sweden | 0.1 | Nuclear power reactors |
Ukraine | 0.08 | Nuclear power reactors |
0.02 | Nuclear fuel cycle facilities | |
United Kingdom | 0.3 | Nuclear fuel cycle facilities |
United States of America | 0.25 | Nuclear fuel cycle facilities |
Noting that the INPRO methodology demands that the NES analysed should demonstrate an improved overall
environmental performance compared to current systems, an assessor should strive for lower dose constraints than
those used today.
Thus, for example, in the case where a level of a radiological stressor of the NES is higher than that in a
comparable current NES or a new stressor exists in the NES analysed, the assessor should set the dose constraints
for a single facility (component) of the NES assessed towards the minimum of the values in Table 12.
This leads to values recommended to be used as INPRO ALs, for the cases when dose constraints are not
defined, as follows:
- A value of 0.08 mSv/a for nuclear power reactors;
- A value of 0.2 mSv/a for all other nuclear facilities of the NES assessed.
Method to set dose constraints in the United Kingdom
The safety assessment principles (SAPs) of the UK Office for Nuclear Regulation contain a set of principles that are structured into 12 separate sections, including, among others, fundamental principles, engineering principles, numerical targets and legal limits [69]. The SAPs include eight fundamental principles that are “founded in UK health and safety law and international good practice” [69]. They embody the requirements for the ALARP concept (see Appendix III) to be applied to radiation exposures resulting from normal operation and to the risks from accidents. The eight fundamental principles are [70]:
(a) “The prime responsibility for safety must rest with the person or organisation responsible for the facilities and activities that give rise to radiation risks.”
(b) “Effective leadership and management for safety must be established and sustained in organisations concerned with, and facilities and activities that give rise to, radiation risks.”
(c) “Protection must be optimised to provide the highest level of safety that is reasonably practicable.”
(d) “Dutyholders must demonstrate effective understanding and control of the hazards posed by a site or facility through a comprehensive and systematic process of safety assessment.”
(e) “Measures for controlling radiation risks must ensure that no individual bears an unacceptable risk of harm.”
(f) “All reasonably practicable steps must be taken to prevent and mitigate nuclear or radiation accidents.”
(g) “Arrangements must be made for emergency preparedness and response in case of nuclear or radiation incidents.”
(h) “People, present and future, must be adequately protected against radiation risks.”
The UK Health and Safety Executive (HSE) risk management philosophy is based on the decision making
process “Reducing Risks, Protecting People” [71]. This defines individual and societal risks to workers and the
public which are so high that they are intolerable, and risks which are so low that they may be considered broadly
acceptable and no further regulatory pressure to reduce risks further would be applied. Between these levels, the
risks must be reduced to ALARP [70, 72].
Licensees are required to assess the average effective dose equivalent (including any committed effective
dose equivalent) to specified classes of persons.
The limits on radioactive discharges are set on the basis of the ‘justified needs’ of the licensees, i.e. the
licensees must make a case that the allowed discharges are necessary to allow safe and continued operation of the
plant.
In setting limits, the Environment Agency, the Scottish Environment Protection Agency and the Northern
Ireland Environmental Agency use monitoring, discharge and plant performance data to ensure that the radiation
exposure of the public as a consequence of the discharges would be less than the dose constraints and limits set by
the UK Government [73]. Currently, these are:
- A single source constraint of 0.3 mSv/a for an individual nuclear installation. Reference [68] explains that in WS-G-2.3 [33] “the experience of numerous countries in establishing dose constraints has been reviewed; the values are mainly between 0.1 and 0.3 mSv, which can be considered a relatively narrow range. Based on this analysis the Guide [33] recommends that as a rule the annual dose constraint should not exceed 0.3 mSv” (see fig. 3 of WS-G-2.3 [33]). If the lower dose of the interval was used as the basic limit (BL), a factor of ten lower value for the basic objective (BO) would mean 10 μSv/a, which is the exemption level shown in fig. 3 of WS-G-2.3 [33], and which is thus fully consistent with the current approach for dose control.
- A site constraint of 0.5 mSv/a for a site comprising more than one source.
- A dose limit of 1.0 mSv/a from all sources of human-made radioactivity including the effects of past discharges but excluding medical exposure.
As a general policy, the limits in discharge authorizations are progressively reduced and are kept close to the levels of the actual discharges.
Concepts, models and tools for assessment of radiological impact on non-human biota
International Commission on Radiological Protection approach
Until 2003, the system for radiation protection of the environment was based on the assumption that the
standards of environmental control needed to protect people to the degree currently thought desirable will ensure
that other species are not put at risk. In Ref. [74], the ICRP acknowledges that this system provided “indirect
protection of the human habitat but that a more comprehensive approach to study the effects on, and thus the
protection of, all living matter with respect to ionising radiation” should be developed. The 2007 recommendations
of the ICRP [34] effectively extend the system of protection to address protection of flora and fauna explicitly.
These recommendations explore the objectives of environmental protection and explain the basis for the proposed
RAP, which is a small set of hypothetical entities that are representative of animals and plants present in different
environments (terrestrial, freshwater and marine) and which form the basis of a structured approach to the
assessment of exposures to, and effects of, ionizing radiation.
The concept and use of RAPs are dealt with in more detail in Ref. [35], which contains information on the
assumed biology, dosimetry and the available effects database for these entities. A range of derived consideration
reference levels (DCRLs) is also proposed for each of the RAPs as numerical guidance for evaluating the level of
potential or actual radiological impacts and as an input to decision making. These values are defined in terms of
bands of doses within which certain effects have been noted, with a focus on those that might have some impact
on the population structures of the animals and plants under consideration. Reference [36] was issued in 2009, and
provided transfer parameters for the set of RAPs.
In 2014, the ICRP published the framework for protection of the environment and how it should be applied
within the ICRP system of protection [37]. The report discusses: the protection of animals and plants (biota) in their
natural environment, and how it can be done with the use of RAPs; their DCRLs, which relate radiation effects to
doses over and above their normal local background natural radiation levels; and different potential pathways of
exposure. It provides information on the different types of exposure situation to which its recommendations apply
and how reference values based on the use of DCRLs can be used to inform on the appropriate level of effort
relevant to different exposure situations. Reference [37] also describes existing types of environmental protection
legislation currently in place in relation to large industrial sites and practices, and the various ways in which wildlife
is protected from various threats arising from such sites.
Overall, these developments represent the latest recommendations and data that can be used for protection
of biota species and are the basis for the Environmental Risk from Ionising Contaminants: Assessment and
Management (ERICA) tool updates.
Environmental Risk from Ionising Contaminants: Assessment and Management tool
Organized between 2004 and 2007, the European Commission project ERICA developed the ERICA
integrated approach to assessment and management of environmental risks from ionizing radiation, with emphasis
on biota and ecosystems. The objectives of the ERICA project have been achieved through the development of
a user friendly assessment tool with risk characterization methodologies coupled with communication strategies
aimed at decision making.
The ERICA project was built partly on the achievements of the Framework for Assessment of Environmental
Impact (FASSET) project, which provided a basic framework for the assessment of the environmental impact
of radionuclides. The FASSET Radiation Effects Database (FRED) has been updated, and a part renamed
FREDERICA is available on-line. ERICA moved towards a tiered assessment approach [75]:
“Using the ERICA reference organisms, Tier 1 will make use of conservative assumptions to derive dose limiting activity concentrations in environmental media (e.g. soil, air or water) for comparing with measured or predicted environmental concentrations around the (proposed) site. The derivation of a screening activity concentration will make use of a number of evaluations of effects data but will be based on threshold values below which effects are unlikely to be observed.
“Tier 2 assessments will predict dose rates to the reference organisms in ERICA and these will be compared to the same dose rate as used to derive the screening level in Tier 1. However it is felt that assessors might find it useful to evaluate the types of effects that may occur in different wildlife groups for the predicted dose rates.…
“Tier 3 assessments will predict dose rates and the assessments will focus on the effects that may be observed within the different wildlife groups.”
Department of Energy graded approach and RESRAD-BIOTA tool
When a judgement based on a comparison with current comparable facilities/environmental conditions/
regulatory requirements is not possible for non-human biota, the INPRO assessor can use the DOE-GA [24], which
is similar to the radiological impact on humans described in Section II.2.
Table 13 shows an overview of the DOE model indicating the parts that should be applied in an environmental
analysis. General screening may serve as tier 1, and site specific screening may serve as tier 2, as specified in
Section II.2 for radiological impact on humans.
Phase* | Implementation |
---|---|
1. Datat assembly | Assemble environmental media data and define evaluation area |
2. General screening | Compare media concentrations with the biota concentration guides |
3(a). Analysis** — site specific screening | Employ site representative parameters and conditions |
3(b). Analysis** — site specific analysis | Employ kinetic/allometric modelling tool |
3(c). Analysis** — site specific biota dose assessment | Employ ecorisk framework |
* - Only phases 1, 2 and 3(a) should be considered for the proposed first application of the method for a simplified environmental analysis in the framework of INPRO assessment.
** - ‘Analysis’ consists of three increasingly more detailed steps of analysis.
The DOE-GA process has been designed in three phases.
Phase 1: Data assembly
This is a data assembly phase in which the evaluation area and its characteristics are defined, and radionuclide concentration data for water, sediments and soil are assembled for subsequent screening [63]. This phase includes the consideration of species and media involved, generic site characteristics in case they are known or postulated, and, because measurements will not (yet) exist, calculated concentrations in air and water as well as in sediments (suspended, bottom and shore/beach sediments).
II.3.3.2. Phase 2: General screening (tier 1)
This is an easy to use general screening method that provides limiting radionuclide concentrations (biota concentration guides, BCGs) in soil, sediments and water such that the dose limits for protection of biota are not exceeded [63].
Phase 3: Site specific screening analysis (tier 2)
This is an analysis phase containing three increasingly more detailed steps comprising site specific screening, site specific analysis and site specific biota dose analysis [63]. This phase is limited to the first step only, and the other steps could be used only for a more mature NES and if some knowledge of the likely sites exists or if generic/typical site characteristics are postulated. The BCGs are very conservative concentration limits for environmental media (water, sediment or soil) defined by the DOE-GA model for a general screening process. The BCGs are calculated in such a way that total doses (i.e. from internal as well as external exposures) received by real biota exposed to these concentrations are not expected ever to exceed biota dose limits. As the DOE-GA model aims at protecting ‘all biota, everywhere’, the BCGs are restrictive, and, in many circumstances, are overly conservative with regard to specific environments [76]. The potential dose rate to a receptor from both internal and external exposures is calculated for the unit concentration of a radionuclide in each medium, i.e. for 1 Bq/kg of sediment for soil and 1 Bq/m3 for water. This potential dose rate is termed dose conversion factor, and is measured in Gy/a per Bq/kg. From the dose conversion factor, the concentration of the contaminant in each medium that will generate a dose limit (DL) can be calculated using a relation of the type [76]:
The dose limits to be used in analysis as ALs are discussed in Section II.3.4. The limits are 10 mGy/d for aquatic animals and terrestrial plants, and 1 mGy/d for terrestrial animals. The total dose conversion factor is made of a part related to external exposure and a part related to internal exposure [76]. When multiple radionuclides are present in multiple environmental media, the screening is based on a summation of the fractions Ci/BCGi for all radionuclides, where Ci is the concentration of the specific radionuclide i, for each medium involved, i.e. water and sediment for aquatic biota, and soil and water for terrestrial biota. To demonstrate compliance with the dose limit, the sum must be less than 1 [24]:
; (6)
BCGs are calculated for several radionuclides i, and implemented in the RAD-BCG Calculator [24] and
RESRAD-BIOTA [77].
Reference [76] notes that empirically based parameters that describe concentrations of contaminants in
an organism relative to the surrounding medium are available for many radionuclides for plant/soil, for aquatic
species/water and, in some cases, for animal/soil or sediment. The advantage of using one of these lumped
factors is that it allows the prediction of tissue concentration based on simple measurements of contamination in
environmental media such as water, sediment and soil.
When the initial (over conservative) screening exceeds the defined dose limits (see Section II.3.4),
non-human biota may still, in reality, receive doses below such limits. This is because actual concentration ratios
for a single radionuclide may range over several orders of magnitude, depending upon various characteristics of
the environment [76]. The more site specific screening phases of the DOE-GA model would allow an analyst to
examine the case with increasingly more accurate representative parameters for the site and receptors.
The environmental analysis should not go very far with details; rather, it should be kept simple, especially
for low maturity NESs. Reference or hypothetical sites may serve for simplified calculations, and assumed
contamination levels may be either estimated on the basis of analogy with similar current conditions (justification
is up to the analyst) or with simplified tools.
The methods of the DOE-GA have been encoded in a simple way, enabling the use of calculation tools such
as the RAD-BCG Calculator [24] (electronic spreadsheets) and RESRAD, in particular RESRAD-BIOTA [77]
(software).
The RAD-BCG Calculator spreadsheets [24, 63] enable the user:
- To use site specific and receptor specific environmental transfer factor parameters (e.g. sediment and soil distribution coefficients, bioconcentration factors) in place of default values;
- To compare radionuclide specific data with radionuclide specific BCGs;
- To verify compliance with the total dose limit, i.e. determine whether the sum of fractions for all radionuclide concentrations/BCG is less than 1.0;
- To apply user specified or regulatory agency specified biota dose limits;
- To modify, when technically justified, the default parameters used in the general screening phase, and to calculate the site specific BCGs using site specific information representing the evaluation area and receptors;
- To modify the radiation weighting factor for alpha emitters;
- To opt into the inclusion of progeny in the calculation of internal dose factors;
- To apply correction factors for the fraction of time that contamination is present in an evaluation area, and for the fraction of time that an organism resides in the contaminated area.
Since 2001, the DOE has been working in partnership with offices of the United States Environmental Protection Agency and the NRC to develop RESRAD-BIOTA, which has wider modelling capabilities (even beyond the current DOE-GA methodology) and a more effective user interface than the RAD-BCG Calculator [63]. The following additional analysis capabilities are incorporated into the code [63]:
- The ability to apply dose conversion factors based on geometries for a variety of organisms;
- Uncertainty and sensitivity analysis for biota dose estimates and associated parameters;
- Improved tabular and graphical presentation of results;
- Capabilities to link and import environmental radionuclide concentration data generated by other radionuclide
transport codes for subsequent use in evaluating doses to biota within RESRAD-BIOTA.
These codes are not further described in detail here because the method for the first stages of application
proposed is quite simple. In addition, details can be easily found in the cited literature. In more advanced stages,
a (generic) site description would be necessary, and specific expertise in site characterization and radionuclide
transfer and transport in different media would also become necessary. However, addressing such issues is beyond
the scope of this publication.
As described above, the DOE-GA provides flexibility and the ability to iterate through the evaluation process.
Parameter values, radiation weighting factors and organism residence times can be modified [63].
Table 14 shows a summary of the DOE-GA for the evaluation of radiation doses to aquatic and terrestrial
biota [24]. Only phases 1, 2 and 3(a) should be considered for the proposed first application of the method for a
simplified environmental analysis. Table 17 shows parameters that could, with technical justification, be modified,
corresponding to each phase of the DOE-GA.
Phase* | Description | Parameters* |
---|---|---|
1. Data assembly | Knowledge of sources, receptors and routes of exposure for the area to be evaluated is summarized. Measured radionuclide concentrations in water, sediment and soil are assembled for subsequent screening. | Size of evaluation area Radionuclide concentration in environmental media |
2. General screening | Maximum measured radionuclide concentrations in an environmental medium (i.e. water, sediment, soil) are compared with a set of biota concentration guides (BCGs). Each radionuclide specific BCG represents the limiting radionuclide concentration in an environmental medium that would not result in recommended dose standards for biota to be exceeded. | Initial general screening using maximum radionuclide concentration: no parameter modifications are allowed |
3(a). Analysis** — site specific screening | Site specific screening using more realistic site representative lumped parameters (e.g. bioaccumulation factors) in place of conservative default values used in the general screening phase. Use of mean radionuclide concentrations in place of maximum values, taking into account time dependence and spatial extent of contamination, may be considered. | Sediment values may be modified, with technical justification, for aquatic system evaluations where only water or only sediment concentration data are available for the screening process |
3(b). Analysis** — site specific analysis | Site specific analysis employing a kinetic modelling tool (applicable to riparian and terrestrial animal organism types) provided as part of the graded approach methodology. Multiple parameters which represent contributions to the organism internal dose (e.g. body mass, consumption rate of food/soil, inhalation rate lifespan, biological elimination rates) can be modified to represent site and organism specific characteristics. The kinetic model employs allometric equations relating body mass to these internal dose parameters. | Exposure area or receptor residence time correction factors for all organism types may be considered For riparian and terrestrial animals the following parameters can be modified: food source; body mass; uptake fraction of radionuclide ingested/ absorbed; biological elimination rate constant of radionuclide exiting the organism; food/soil intake rates, inhalation and soil inhalation rates and supporting parameters; water consumption rate; maximum lifespan; allometric equation provided |
3(c). Analysis** — site specific biota dose assessment | Actual site specific biota dose assessment involving the collection and analysis of biota samples. The dose assessment would involve a problem formulation, analysis and risk characterization protocol consistent with the widely used ecological risk assessment paradigm. | Design, collection and direct analysis of environmental media and biota |
* - Parameters that can be modified. The RAD-BCG Calculator provides capabilities to modify the dose limits for aquatic and terrestrial organisms, to modify the relative biological effectiveness weighting factor for alpha emitters and to deselect inclusion of energies for progeny of chain decaying nuclides with regard to internal dose conversion factors. These default values are to be used in dose evaluations conducted for DOE sites.
** - ‘Analysis’ consists of three increasingly more detailed steps of analysis.
Limits for radiation protection of non-human biota and their application for environmental assessments
In the following, the corresponding limits for radiation protection of non-human biota (plants and animals)
are presented to be used in the case when simplified analysis needs to be performed within the framework of
INPRO assessment in the area of environmental stressors.
Increasing attention has been paid to radiological protection of non-human environmental components,
in particular biota, independently from protection of humans. Avoiding measurable impairment of reproductive
capability is deemed to be the critical biological end point of concern in establishing the dose limits for aquatic
and terrestrial biota [41]. Other aspects being considered are individual lethal doses, genetic modifications and
biodiversity.
The DOE [78] has defined a radiation dose limit for the protection of aquatic organisms [79], and has
considered (absorbed) dose limits for terrestrial biota [63, 80]. The limits for absorbed dose, below which no
deleterious effects on populations of aquatic and terrestrial organisms have been observed, as discussed by the
National Council on Radiation Protection and Measurements (NCRP) [81] and the IAEA [41, 82], are:
- A limit of 10 mGy/d (1 rad/d) for aquatic animals, from exposure to radiation or radioactive material releases into the aquatic environment;
- A limit of 10 mGy/d (1 rad/d) for terrestrial plants, from exposure to radiation or radioactive material releases into the terrestrial environment;
- A limit of 1 mGy/d (0.1 rad/d) for terrestrial animals, from exposure to radiation or radioactive material releases into the terrestrial environment.
For implementation of these proposed limits for protection of biota, the DOE has developed methods, models and guidance within a graded approach for evaluating radiation doses to non-human biota [24, 76, 83]. These methods are briefly described in Sections II.3.1–II.3.3.
Study of radiological impact on non-human biota in the United Kingdom
As an example of application of simplified methodologies for the estimation of effects of radionuclides to
non-human species, during the Environment Agency’s re-examination of 2000–2002 radioactive discharges and
disposals from the Sellafield site, a specific study was performed [84]. Internal dose rate conversion factors for
non-human biota were determined using a relatively simple and conservative method [84]. Utilizing modelled and
measured radionuclide activity concentrations, these dose rate conversion factors were employed to estimate whole
body doses. The highest doses to non-human biota due to measured and predicted concentrations were estimated
to be at least an order of magnitude below the level of 1×10−3 Gy/d suggested by the IAEA [41] as a threshold
for chronic exposure to radiosensitive species in the terrestrial environment, below which measurable detrimental
effects on populations are unlikely to be observed [84]. It is concluded in Ref. [84] that the result would suggest
that measures taken to apply “best practicable means” may have been unnecessarily conservative. However, one
order of magnitude may cover the added higher uncertainties of discharged amounts, dispersion patterns, biota
involved and site characteristics.
In 2001, the Environment Agency conducted a review of available data on radiation effects on biota [85] and
concluded that it is unlikely there will be any significant effects at:
- Chronic dose rates of 400 μGy/h (= 10 mGy/d = 1 rad/d) for populations of freshwater and coastal organisms;
- Chronic dose rates of 400 μGy/h (= 10 mGy/d = 1 rad/d) for terrestrial plant populations;
- Chronic dose rates of 40 μGy/h (= 1 mGy/d = 0.1 rad/d) for terrestrial animal populations.
These findings are the same as those from the DOE and are largely consistent with the findings and biota dose
recommendations of the NCRP [81], the IAEA [41] and UNSCEAR [38].
Additionally, the Environment Agency concluded that it is unlikely that there will be any significant effects
at [85]:
- Chronic dose rates below 1000 μGy/h (25 mGy/d = 2.5 rad/d) for populations of organisms in the deep ocean.
Sections II.4.1–II.4.5 briefly describe international activities (and their background) regarding radiation dose limits for non-human biota. This introductory information can be used when further elaboration on the ALs for doses to the reference biota species is needed.
International Commission on Radiological Protection
Radiological protection has traditionally focused on the protection of humans. In the past, the ICRP has taken the position that “the standards of environmental control needed to protect man to the degree currently thought desirable will ensure that other species are not put at risk” [86]. This position has now changed [34, 74], and environmental effects, i.e. the impact of ionizing radiation specifically on the environment, and protection of the environment against its harmful effects is now part of the development activities of the ICRP [35, 36].
International Atomic Energy Agency
Between 1986 and 1992 [82], the IAEA examined the validity of the implicit assumption that protecting
humans will also protect the environment [86], and published its conclusions for the case of radioactive releases to
the terrestrial and freshwater environments, as well as solid waste disposal underground [41], and for the of case
marine disposal of radioactive waste [87]. The conclusion in Ref. [41] was that there is no convincing evidence
from the scientific literature that chronic radiation dose rates below 1 mGy/d will harm animal or plant populations.
This should be about the upper value of the range for doses to biota in areas where critical groups live exposed to
radiation, where the limit of 1 mSv/a applies. In the aquatic environment, it would appear that limiting chronic dose
rates to 10 mGy/d or less to maximally exposed individuals in a population would provide adequate protection to
the population.
However, reliance on human based radiological protection may not be adequate for all possible conditions,
e.g. in situations where humans are not present or where they live far away from the sources of radionuclides.
Environmental Modelling for Radiation Safety
From 2003 to 2007, the IAEA conducted the Environmental Modelling for Radiation Safety (EMRAS)
programme [88]. This focused on the development, comparison and testing of environmental assessment models
for estimating radiation exposure of humans and radiological impacts on flora and fauna due to actual and potential
releases of radionuclides to terrestrial and aquatic environments. EMRAS was followed by EMRAS II, which
continued some of the work of previous international exercises in the field of radioecological modelling and
focused on areas where uncertainties remain in the predictive capability of environmental models.
Modelling and Data for Radiological Impact Assessments
The IAEA programme on Modelling and Data for Radiological Impact Assessments (MODARIA) was
planned for 2012–2015. The general aim of the MODARIA programme was to improve capabilities in the field
of environmental radiation dose assessment by means of: acquisition of improved data for model testing; model
testing and comparison; reaching a consensus on modelling philosophies, approaches and parameter values;
development of improved methods; and exchange of information. MODARIA has continued some of the work of
previous international exercises in the field of radioecological modelling, and focused on areas where uncertainties
remain in the predictive capability of environmental models. These previous international exercises include the
Biospheric Model Validation Study (BIOMOVS) and BIOMOVS II, initiated by the Swedish Radiation Authority
Biosphere Modelling and Assessment (1996–2001), EMRAS (2003–2007) and EMRAS II (2009–2011).
Coordination Group on the Radiation Protection of the Environment
The international Coordination Group on the Radiation Protection of the Environment (CGRPE)
was established by the International Plan of Activities on the Radiation Protection of the Environment in
September 2005 [89]. It serves as a mechanism to facilitate the coordination of activities among international
organizations by reviewing their ongoing work related to protection of non-human species. The IAEA organizes the
Secretariat of the CGRPE.
Database of Discharges of Radionuclides to the Atmosphere and the Aquatic Environment
The IAEA Member State Database on Discharges of Radionuclides to the Atmosphere and the Aquatic
Environment (DIRATA) contains the annual records submitted on a voluntary basis by IAEA Member States.
DIRATA also includes the historical discharge records, collected by UNSCEAR, the European Commission and
other international and national organizations.
United Nations Scientific Committee on the Effects of Atomic Radiation
In 1996, UNSCEAR summarized and reviewed information on the responses to acute and chronic radiation of plants and animals, as individuals as well as populations [38]. The conclusions were consistent with the findings and recommendations made earlier by the NCRP and IAEA concerning biota effects data and appropriate dose rate CR for protection of biota populations, i.e. 10 mGy/d for aquatic animals and terrestrial plants, and 1 mGy/d for terrestrial animals. Since 2005, UNSCEAR has been developing developing a new scientific annex that addresses the effects of radionuclides released to the environment as well as a methodology for dose assessment for biota.
International Union of Radioecology
The International Union of Radioecology (IUR) poses the problem of protection against ionizing radiation
simultaneously for both biota (environment) and humans, i.e. it deals with biota protection by a combination of
anthropocentric and ecocentric approaches.
The IUR’s main role is based on science, promoting its advancement, the dissemination of its knowledge
and the communication of this to society. As such, the IUR does not set out to promote particular standards or
regulations, but to contribute to the international effort aimed at developing a general framework that will allow
such management tools to be derived from a sound scientific basis. A further strength of the IUR is the capacity
of the multidisciplinary scientific community gathered within the IUR to provide expert, informed, up to date
and independent scientific advice, incorporating the knowledge from wider environmental fields not focused
uniquely on radioactivity. The ultimate goal of the IUR is to ensure that the radioprotection of both people and the
environment are considered with a scientifically sound, balanced and appropriately precautionary approach that
permits sustainable development and technical innovation. The basic document governing the IUR policy in the
area of radiation protection is presented in Ref. [90].
Advisory Committee on Radiation Protection
In 2002, the Advisory Committee on Radiation Protection (ACRP) provided recommendations to the Canadian Nuclear Safety Commission concerning dose rate limits for protection of biota. The ACRP recommended a generic dose rate criterion in the range 1–10 mGy/d. The ACRP specified that this dose rate CR is based on population level effects, and, given the current state of knowledge and consensus views of radiation effects on biota, represents the level at which ecosystems will suffer no appreciable deleterious effects. The criterion is specified in terms of daily dose to prevent cases for which this dose rate criterion would be received in a few days [91] (as reported in Ref. [24]).
Calculation of impacts of chemical stressors on humans and the environment
There are currently no internationally agreed computer models to calculate the impacts (caused by the release)
of toxic chemicals on humans and the environment. Specific computer models can be presented on the web site
of the national environmental protection agency that uses these tools (e.g. models used by the US Environmental
Protection Agency are presented in Ref. [92]).
In the European Union, a feasibility study was performed in 2007 that identified existing models to calculate
the environmental concentrations of chemicals from emissions [93]. The study identified a comprehensive list of
existing models to calculate the environmental impacts of chemical stressors, most of which are available on-line.
It verified the status of validation and general acceptance of these models.
RESRAD-Chem
RESRAD is a series of computer models designed by Argonne National Laboratories to estimate primarily
radiation doses and risks from residual radioactive materials. One code of this family is called RESRAD-Chem,
and it presents a model for evaluation of sites contaminated with hazardous chemicals [94].
RESRAD-Chem calculates cancer incidence risks and hazard indices to an on-site exposed individual and
derives site specific soil cleanup CR for hazardous chemicals. It follows the United States Environmental Protection
Agency Risk Assessment Guidance for Superfund (RAGS). It considers nine exposure pathways: inhalation of dust
and volatiles, ingestion of plant foods, meat, milk, soil, aquatic food and water, and dermal absorption from soil
and water contact.
Regulatory limits of chemical stressors on humans and the environment
As already mentioned in Section 2.4.2, there are no internationally agreed regulatory limits for chemical
stressors. Thus, an INPRO assessor performing an assessment of the environmental impact of release of chemicals
has to apply the regulatory limits of the country where an NES facility is planned to be installed.
There are, however, some international guidelines available, such as the following World Health Organization
(WHO) guidelines for limits of toxic chemicals stressors on humans:
- Air Quality Guidelines for Europe [95];
- Guidelines for Drinking-water Quality [96].
These publications establish guideline values for chemicals in air and drinking water which are of significance to health. The report of the INPRO assessment of the planned NES of Belarus [97] presents an application of national standards (limits) on the release of toxic chemicals from a nuclear power plant.
Representative examples of environmental studies
In the following, in addition to the simplified methods of analyses [23, 24] discussed in Sections II.2 and
II.3, selected examples of environmental studies are presented. These studies could be used to identify comparable
current facilities for the NES facilities to be assessed and to extract an appropriate list of stressors (primarily
radiological), a measure of the levels (and impacts) of the stressors and, in some cases, the applicable regulatory
standards.
An incomplete overview of radiological emissions and doses involving estimations performed for milling
facilities, MOX fuel fabrication and fuel reprocessing is reported in Ref. [30] and is reproduced here for illustration
in Tables 15–18.
Radionuclide | Normalized release* (GBq/(GW(e)∙a)) | Normalized collective effective dose* (man Sv/(GW(e)∙a)) | ||||
---|---|---|---|---|---|---|
Mill | Mill tailings | Mill | Mill tailings | |||
In operation | Abandoned | In operation | Abandoned | |||
Pb-210 | 0.02 | 0.00002 | ||||
Po-210 | 0.02 | 0.00002 | ||||
Rn-222 | 3000 | 20000 | 1000** | 0.045 | 0.3*** | 150**** |
Ra-226 | 0.02 | 0.00001 | ||||
Th-230 | 0.02 | 0.0006 | ||||
U-234 | 0.02 | 0.003 | ||||
U-238 | 0.02 | 0.003 | ||||
Total | 0.05 |
* - Normalized emissions in liquid effluents (0.01 for Pb-210 and Th-230; 0.02 for Ra-226; 0.3 for U-234 and U-238) contribute negligibly to the collective dose.
** - Annual activity released; the rate of activity is assumed to remain constant over more than 10 000 years.
*** - Dose commitment corresponding to a five year release.
**** - Dose commitment corresponding to a 10 000 year release.
Type of emission | Value |
---|---|
Annual atmospheric emissions (Bq/a) | 107 - 108 |
Normalized annual emissions (Bq/(GW(e)∙a)) | 107 - 108 |
Effective dose | Existing plants (experience) | Modern plants (design) |
---|---|---|
Annual effective dose per monitored worker (Sv/a) (average) | 0.007 | 0.003 |
Collective effective dose per produced mixed oxide tonne (man Sv/t) | 0.07 | 0.005-0.020 |
Normalized collective effective dose (man Sv/(GW(e)∙a)) | 2.0 | 0.15 - 0.60 |
Time period | Monitored workers (thousands) | Annual collective effective dose (man Sv) | Annual effective dose per monitored worker (mSv) |
---|---|---|---|
1975 - 1979 | 0.1 | 0.36 | 4.0 |
1980 - 1984 | 1.0 | 2.4 | 2.3 |
1985 - 1989 | 4.0 | 5.7 | 1.4 |
Values of actual annual emissions from currently installed nuclear facilities can be found in several publications
(e.g. Ref. [98]). Annex C of Ref. [98] includes mining and milling (emissions per tonne of produced uranium, using
a model mine and mill), uranium enrichment, fuel fabrication, nuclear reactor operation, fuel reprocessing and
solid waste disposal. For power plants, power production and emission data in recent years are included for noble
gases, tritium, 131I and particulates to air, and tritium and other radionuclides to water. Reference [98] also reports
the specific normalized collective effective doses in terms of the units man Sv/GW∙a.
The normalized collective effective dose of 7.5 man Sv/GW∙a is calculated in Ref. [98] for mill tailings of
radon releases integrated over 10 000 years. Incidentally, the releases to groundwater from low and intermediate
disposal in shallow depositories of waste from reactor operation produce 0.5 man Sv/GW∙a, mainly from 14C.
A review of five year annual emissions from individual nuclear power plants in the European Union is reported
in Ref. [99]. Data were taken from plant annual reports and comprise, for airborne emissions: the noble gases,
tritium, 131I and other beta and gamma emitters; for emissions to water: tritium, other beta and gamma emitters
(including spectra of individual radionuclides when available) and alpha emitters when available. Reference [99]
also reports discharges from reprocessing sites in Europe, La Hague and Marcoule (France), and Sellafield and
Dounreay (United Kingdom), which include aerial discharges of tritium, total beta and gamma emitters (without
tritium), 14C, 85Kr, iodine isotopes and total alpha emitters (individual radionuclides are also given for Dounreay
and Sellafield). Data on liquid discharges from reprocessing sites include tritium, total beta and gamma emitters
(without tritium), 14C and total alpha emitters. Liquid discharge spectra are also included for the four sites. Portions
of discharge limits are indicated for individual species or groups of radionuclides.
Emission data as well as doses to critical groups can be found in annual environmental reports of several
nuclear facilities, e.g. for reprocessing at La Hague, the annual report [55] includes radioactive, as well as
non-radioactive emissions, and the site authorization levels for all discharge classes. Airborne radioactive emissions
given in Ref. [55] are tritium, iodine, noble gases including 14C, 85Kr, other beta and gamma emitters, as well as
alpha emitters.
Reference [100] provides data on radioactive emissions to the sea: tritium, iodine, 134Cs, 137Cs, 14C,
60Co, 106Ru and 90Sr, other beta and gamma emitters, as well as alpha emitters. Non-radiological emissions to
air comprise: CO, NOX, SO2 and particulates. Reference [101] reports volatile organic compounds, ammonia,
calcium, hydrogen chloride, hydrogen fluoride, silicon and sodium, and the heavy metals iron, nickel, titanium
and vanadium. Non-radiological emissions to water comprise: aluminium, ammonia, barium, cadmium, chemical
oxygen demand, chromium, cobalt, fluorine, hydrazine, iron, lead, manganese, mercury, nickel, nitrate, nitrite,
phosphorous, sulphur, tributylphosphate, zinc and zirconium. Annual amounts of industrial and hazardous wastes
are presented. In addition, the demands of chemicals (sodium hydroxide, nitric acid, formaldehyde), water, fossil
fuels and electricity are provided, which can be useful for life cycle accounting of secondary material flows [102].
The assessment of radiological impacts, in terms of biota dose rates and their related potential biological
effects on marine biota arising from radioactive discharges to the sea (as liquid effluents) of the La Hague facility
can provide a part of the necessary input data for the INPRO assessment of a planned NES facility [103, 104]. In
Ref. [103], a representative set of local marine biota is selected for assessment. The guidance values are based
on Refs [38, 41], and on a review of the database developed in the FASSET project [105]. The derived generic
guidance values are similar to those published in the MARINA II study on radioactive discharges into north
European marine waters, concentrations of radionuclides in the environment and assessment of their impact [106].
The study provides dose rate results for selected marine biota categories for the coastal areas of La Hague and
Sellafield. MARINA II also includes generic guidance values for the protection of marine biota [104, 106]. The
estimated marine biota dose rates are low (by at least two to three orders of magnitude) compared with the lowest
generic guidance values for the protection of populations of marine biota [103].
A summary of an environmental assessment of Bruce, a Canadian nuclear power plant, can be found in
Ref. [107], which also includes non-radioactive stressors that are, however, producing negligible impacts.
A generic comparative study has been performed by the OECD Nuclear Energy Agency (NEA) on the
radiological impact of spent nuclear fuel options [108]. The options considered were a pressurized water reactor
(PWR) with an open (once through) uranium fuel cycle and the same PWR with monorecycling of uranium fuel.
Generic release rates of radionuclides of all fuel cycle facilities including the power plant can be found in Ref. [108].
Comprehensive environmental studies
The nuclear fuel cycle has been the object of a few life cycle assessment (LCA) studies focused on greenhouse
gases or energy expenditures, but relatively few comprehensive studies have been published on current nuclear fuel
cycles, and even fewer on future NESs. Known published examples of comprehensive studies on current operating
NESs are given below.
Within the Externalities of Energy (ExternE) project of the European Commission, the French nuclear fuel
cycle was evaluated. All stages were included in the assessment, from mining uranium ores through to waste
disposal. The general methodology adopted in the study was the ‘impact pathway analysis’, modelling the path
from discharges into the environment through dispersion and deposition to potential doses to humans. The damages
(collective doses to workers and the public) were converted to costs, using a single framework devised for all the
fuel cycles considered in the ExternE project [109–111].
The Swiss LCA study on current (reference year 2000) European energy systems [112–114] included the
nuclear fuel cycles for PWRs, boiling water reactors (BWRs) and country specific LWR mixes [102, 113]. Both
open and closed fuel cycles (one time reprocessing of spent fuel, at equilibrium) were analysed. All stages of
the front and back ends were included. Approximately a thousand elementary environmental flows (requirements
as well as emissions) per unit of net electricity produced at the power plant are available in the ecoinvent LCA
database, described in Section II.6.2. Known examples of comprehensive environmental assessments of possible
future nuclear fuel cycles are given below.
An extrapolation of LCA data of currently installed technology (Swiss LWR) to new designs (AP600 and
advanced BWRs) was performed in Ref. [115]. Also taken into account after a priority analysis were: improvements
in mill tailing management; centrifuge/atomic vapour laser isotope separation enrichment plants substituting
the units based on the gaseous diffusion process once they were phased out; and reduction of waste volumes at
reprocessing. A semistatic approach was defined to calculate cumulative burdens at discrete time points.
An assessment of five different scenarios (once through, single plutonium recycling, plutonium
multirecycling, 45% thermal 55% breeder, and breeder burning neptunium, americium and copper homogeneously
mixed with plutonium) within the twenty-first century in the French context was published in 2004 [116]. The EPR
and European fast reactor types were modelled. Conventional emissions and the health effects of ionizing radiation
(local impacts) were analysed. Application of LCA, covering the global environmental analysis part of the study,
actually focused on a few emission species to air, was complemented by the assessment of local and regional
radiological impacts on humans in terms of annual doses, and by the inventorying of nuclear materials using the
material flow accounting tool COSI.
A project of the European Commission addressed future technologies up to the year 2050, integrating LCA
with external cost estimation and an optimization energy economy code (Times-MARKAL). The nuclear industry is
represented with Generation III and Generation IV power plants, in addition to fossil fuel and renewable technology
power plants. A multicriteria decision assessment (MCDA) methodology will be used, as well as external costings
for comparison and ranking of the technology options.
Life cycle assessment ecoinvent database
The commercial, centralized, web based LCA database ecoinvent was developed and implemented by the
Swiss Centre for Life Cycle Inventories and supported by Swiss Federal Offices. It has been available on-line since
September 2003.
The aim of developing the centralized LCA ecoinvent database was to achieve consistency throughout
different life cycle inventory (LCI) databases maintained by the participating organizations. The sectors included
are: energy systems (Paul Scherrer Institute); materials and metals, waste treatment and disposal (Swiss Federal
Laboratories for Materials Testing and Research); transport systems and chemicals (Swiss Federal Institute of
Technology in Zurich); and agricultural products (Swiss Federal Research Station for Agroecology and Agriculture).
About 2750 individual processes (datasets) have been modelled and about 1000 elementary environmental flows
inventoried, including emissions to air, water, soil, solid wastes, land use, and biotic and abiotic resources. The
environmental cumulative burdens associated with the processes are integrated based on an algorithm, thus
reflecting the interactions of industrial activities within an economy system. In addition to LCI, ecoinvent also
includes the results of LCA, using current methods developed by different organizations [117].
The assessed energy systems, constituting about half of the processes available in the database, include
electricity and heating systems [112, 113]. Electricity transmission and distribution, as well as country specific
production and supply mixes, have also been modelled. Fossil fuel, nuclear and renewable energy systems
associated with Swiss and European power plants, boilers and cogeneration plants have been assessed, reflecting
conditions around the reference year 2000.
The nuclear fuel cycles modelled in ecoinvent are those associated with power generation at LWRs currently
installed in the Union for the Co-ordination of Transmission of Electricity (UCTE) [102, 114]. The focus was on
two 1000 MW units operating in Switzerland: Gösgen PWR and Leibstadt BWR. The conditions of the fuel cycles
for the Swiss reactors were assessed, modelled and extrapolated to France, Germany and the UCTE (which can
be assumed to be representative of LWRs in western Europe). In addition to describing the nuclear cycle as such,
the assessment served the estimation of cumulative environmental burdens of European electricity mixes, along
with other electricity systems in ecoinvent. The stages separately modelled were: mining, milling, conversion,
enrichment (diffusion and centrifuge), fuel fabrication, PWRs, BWRs, national mixes of PWRs and BWRs,
reprocessing, spent fuel conditioning, interim storage, low level radioactive waste depository and geological final
repositories.
Appendix III
BASIC CONCEPTS FOR OPTIMIZING MANAGEMENT OPTIONS FOR REDUCTION OF ENVIRONMENTAL IMPACT
As low as reasonably practicable
Concept
The concept of ALARP (economic and social factors taken into account) is used to define an AL for several INs in different areas of the INPRO methodology, especially for the environment and waste management.
The concept is illustrated in Fig. 5. As shown, the risk (symbolized by the triangle) is divided into three
regions: a broadly acceptable region, a tolerable region where a process for ALARP has to be used and an
unacceptable region.
As a first step of the ALARP concept to be applied within the INPRO methodology, the boundary values of
these three regions have to be determined: the boundary between the tolerable and the unacceptable regions, usually
called a basic limit (BL); and the boundary between the tolerable and broadly acceptable regions, sometimes called
a basic objective (BO). The next step is to confirm that the value of the indicator of an NES is within the ALARP
region that is below the BL. The last step is to confirm that in an optimization analysis, all measures to reduce
the specific risk have been taken into account by the designer up to a level where the costs for these measures
would have become ‘grossly disproportional’ to the benefit gained. It is important to note that, in the case when
the indicator of an NES has a value in the broadly acceptable region below the boundary BO, no further effort is
necessary to fulfil the ALARP concept.
BL and BO values may be specified for specific indicators in national regulations or as an outcome of an
EIA, or it may be necessary to infer such values from other evidence such as: licence conditions; actions planned,
under way or completed to remediate an existing situation or improve a given practice; presentations at national
or international conferences; publications in peer reviewed journals, the IAEA Safety Standards Series and other
IAEA publications; and the work of other organizations such as the ICRP, the NEA and the European Commission.
When a BL or a BO is deduced from such evidence, the rationale for doing so needs to be clearly stated to ensure
transparency. While national and international consensus may not exist and may be slow to emerge, suggesting a
value for a BO and the associated rationale would be expected to make a valuable contribution to the process of
national and international consensus building.
Application of the ALARP concept by a technology developer
The flow chart in Fig. 6 exemplifies the procedure that a technology developer (designer) could follow
to confirm compliance of an NES under development with the INPRO methodology CR3.1 in the area of
environmental stressors (optimization of the measures to reduce environmental impact).
The quantity, optimized in an ALARP analysis, could be an individual stressor level, a weighted combination
of stressor levels (e.g. estimated dose) or some other risk related function of the system parameters. The values of
BL and BO are to be set on the basis of expert judgement, best technology available, regulatory clearance levels or
other information.
The depth that the ALARP analysis can reach depends on the maturity level of the NES assessed. For early
designs that are still at the conceptual stage, the optimization process could be guided by establishing a figure of
merit for acceptable limits and restrict the ALARP procedure to stressors (radioactive as well as non-radioactive,
if relevant) that have been identified as being the most significant or of the highest priority. Further reductions
of the number of stressors could be accepted only if they have been appropriately justified by the designer, with
evidence provided in the analysis report.
The ALARP concept might be applied to the whole NES under development, for which adequate BL/BO
values would need to be defined. In the case when the optimization occurs both at the individual facility (component)
level and at the whole system level, care needs to be taken to ensure that double counting does not occur. As an
example, in addition to a BL and a BO on 14C emitted from a reactor or a reprocessing facility, a BL and BO for the
aggregated 14C from the entire NES under development, including reprocessing and waste treatment facilities, can
also be set (see also the discussion below on the reduction of collective doses).
In the following, the proposed approach for each facility (component) is described in more detail, as it
corresponds to the way that ALARP is mostly applied in current practices.
If the analysis is performed in several stages for addressing successive levels of maturity of the NES being
developed or for considering possible changes in the regulations of the Member State or in international standards,
the BL and BO values might also need to be redefined, and the ALARP analysis may become more refined and
complex.
If the NES being developed is just at its conceptual stage, the ALARP demonstration may be reduced to a
discussion or a series of statements (illustrations) aimed at showing that modifications to the system that could
reduce these stressors have been considered, and that these stressors cannot practicably be reduced further. At the
very least, the developer (designer) should acquire evidence that sufficient thought has gone into evaluating the
potential of adjustments in the NES facilities and processes to reduce environmental effects. The descriptive
demonstration, adequately reported in the analysis report, should be finalized in the following phases of design
development. Reference [72] explains that the requirement for risks to be ALARP “is a requirement to take all
measures to reduce risk where doing so is reasonable. In most cases this is not done through an explicit comparison
of costs and benefits, but rather by applying established relevant good practice and standards.”
Hence, it is expected that if an analysis of more refined stages of the design process is performed, this should
verify ALARP for the same component(s)/stressor(s), and, if the design is changed, track the reasons for technical
modifications in relation to the previous ALARP analysis.
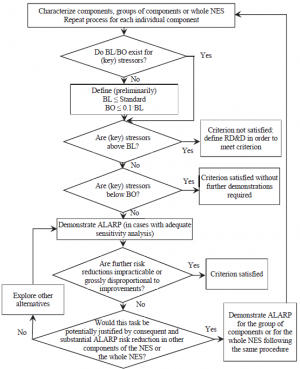
If a facility, or the whole NES, is at a more advanced state of development, ALARP may require the application
of tools for aiding in decisions such as CBA or MCDA [72, 118]. It may happen that ALARP implementation in a
given facility may be challenging (stressors must be below BL for licensing) because of compensating effects across
the spectrum of stressors of the facility itself or group of facilities of the NES. Reference [72] notes that “where
there are several risks which interact, whether arising from a single hazard or from different connected hazards,
there may be a need for balancing to achieve the best overall solution.” In this case, the developer (designer) should
demonstrate and document that ALARP is fulfilled at a higher level by analysing groups of components or the
NES as a whole (see Fig. 6). A sensitivity analysis may be useful to show the robustness of the adopted technical
solution by varying key parameters (see the discussion below on the proportion factor between costs and risks).
CBA and/or MCDA need to be applied considering all aspects within the boundary of the assessment.
With reference to Fig. 6, what ‘gross disproportion between costs and risk reduction’ means for application,
no algorithm has been defined by the risk and safety authorities adopting ALARP, rather only a general guidance
for judgement to allow flexibility on a case by case basis. For current technology, ALARP involves consideration
of good practices [52]. However, this may change owing to innovation, which is improving the degree of control,
cost changes or knowledge about hazards [72]. For new technologies in an NES, evolving good practices could be
followed to start with, and then consideration given to whether there are alternative processes or procedures that
could be performed to reduce the risk on the basis of first principles to compare the risk with the sacrifice involved
in further reducing it. In many instances, such ‘first principles’ comparisons can be carried out qualitatively,
i.e. using common sense or exercising professional judgement or experience [52]. In the case when the costs are
definitively very high and the reduction in risk is just marginal, then ALARP is likely demonstrated, and no further
improvements need to be designed or implemented. Conversely, in most cases where the improvements identified
are simple or cheap to implement and the risk significantly reduced, these improvements are required, and ALARP
can be considered to be achieved without further analysis [52].
For less clear cut situations, which are most likely for innovative technologies, a more formal CBA may
provide additional insight to help to arrive at a judgement. In a CBA, both risk and sacrifice are converted into
monetary terms. In a standard CBA, the rule applies that the measure should be adopted if benefits outweigh costs.
However, in ALARP judgements, the measure has to be adopted unless the sacrifice is grossly disproportionate
to the risk. Therefore, “the costs can outweigh benefits and the measure could still be reasonably practicable to
introduce. How much costs can outweigh benefits before being judged grossly disproportionate depends on factors
such as how big the risk is to begin with (the larger the risk, the greater can be the disproportion between the cost
and risk)” [52].
Although individual doses to members of the critical groups are used for establishment of standards, hence
of BL and BO values, for the CBA, the total social costs of all emissions (radiological as well as non-radiological)
have to be taken into account. Therefore, it is the collective dose (see Appendix IV) that should be used. The scope
of the analysis depends on the choice by the developer (designer) of the consistency within the analysis. Ideally,
externalities should be evaluated considering the LCA of the entire cycle, thus including secondary burdens from
flows of material and energy into the system. However, in the case when the options to be addressed in the ALARP
procedure would not meaningfully change the secondary contributions in relative terms, the analysis can be
restricted to the effect of primary (or direct) burdens only. Studies of external costs for current nuclear technologies
can be found in Refs [110, 115, 119, 120].
The ALARP process should also take into account risks that may affect future generations, as they may stem
from radioactive waste management and decommissioning. Reference [72] notes that “risks should be assessed in a
holistic manner and not restricted to part of the overall time period or part of a process.” Further elaboration points
out that: “Although it could be argued that the next few generations may gain some indirect benefit, the uncertainty
of how they will view the risks left to them (and indeed the uncertainty of any benefits further into the future)
argues for a precautionary approach and hence a particularly stringent demonstration that risks are indeed ALARP.”
Considering that the demonstration of long term sustainability for an NES is the ultimate objective of an INPRO
assessment, the requirement of consideration of effects into the future should be even stronger when applied to an
NES under development.
The following information, although referring to the ALARA approach, may also be of interest for ALARP.
The DOE recommends that for the purpose of comparing ALARA alternatives for operational systems, the lifetime
of the facility generally is a basis for truncating collective dose estimates, temporally [118]. However, where
cleanup, restoration and waste management activities are necessary, the time frame for the analysis can be much
longer. In particular, for long lived radionuclides, integration times may be determined by the uncertainties in
scenarios and by the physical parameters affecting dose rates. The DOE states that, typically, it is only appropriate
to conduct quantitative comparisons for a few hundred years or less. Although evaluating doses for periods of
up to a thousand years may provide useful information, periods beyond this length of time should not be used in
quantitative ALARA assessments [118]. However, although this position may be adopted for an analysis, provided
it is justified by the nature and amounts of the releases, consideration of longer integration times may be useful in
other parts of the analysis.
Reference [72] defines that: “The safety benefit of the improvement is the incremental difference in risk
between the two estimates in terms of the detriments (i.e. all the adverse consequences) and their likelihoods,
summed over the remaining life of the facility.” This includes detriments from normal operation such as radiation
exposures of workers and the public; detriments from accidents which again includes radiation exposures to
the public and workers but also includes other detriments such as the need to decontaminate areas, evacuation,
relocation and food restrictions. Valuation of detriments is a subject of much discussion and research [72].
The UK HSE [71] uses £1.5 million (2009) for the value of preventing a statistical fatality. In the case of
death caused by cancer, the HSE takes the view that people are prepared to pay a premium. Thus, a higher value,
such as twice that defined above, should be used. It is suggested that this higher value is also appropriate for all
radiation deaths.
In the European study ExternE [109, 120], the value for average social (or external) costs in Europe attributed
to any cancer, whatever its origin and outcome (fatal or non-fatal), is €2 million. In the case where an assessment is
performed for a different country, if ‘willingness to pay’ (WTP) estimates are lacking, values established in Europe
or the United States of America can be adjusted to specific conditions using the ratio of the purchasing power
parity gross national product per capita. The adjustments are based on the assumption that there is an ‘elasticity’ of
WTP with respect to real income. An example of the application of this approach to the assessment of electricity
scenarios (including nuclear) for the Shandong Province, in China, can be found in Ref. [121].
Use of external cost calculations implies verification, to the latest available information on damage
functions and external costs in publications, and detailed reporting on the assumptions/options used or possible
modifications/adaptations.
The following applies to ALARA as implemented in the United States of America. However, the information
may also be useful for ALARP assessments. For the costs associated with units of doses, the linear relationship of
health effects with dose is postulated, thus making the monetary value independent of the magnitude of individual
doses. Several rationales have been adopted for the estimation of the monetary value of a unit of collective dose or
other effects of non-radioactive substances, which can be found in the literature. The use of WTP, i.e. the willingness
to commit resources to avoid the negative impact, is considered here. As an example of application, in 1995, the
NRC [122] re-evaluated the monetary equivalent value and issued regulatory analysis guidelines that recommend
US $2000/man rem (US $200 000/Sv) as the current monetary equivalent value for converting collective dose
to US dollars [118]. The DOE accepted the NRC recommendation, and, because of the uncertainty in the values
used in ALARA analyses, further elaborated that detailed ALARA evaluations use the range for comparing
alternatives. The DOE evaluations to support ALARA analyses apply monetary equivalents for a man rem in the
range US $1000–6000 with a nominal value of US $2000 [118, 123]. Assuming that one man rem corresponds to
a potential risk of 5 × 10−4 radiation induced fatal health effects (generally cancers) for the population, the range
recommended by the DOE would correspond to a range of US $2 million to US $12 million per hypothetical
radiation induced cancer death averted [118].
In cases where, in addition to health (and environmental) risks and costs of measures to reduce them, several
other non-health detriments are identified, these should also be included in the analysis with societal costs when
appropriate and feasible. These effects can be physical (e.g. waste heat discharge, noise or damage from potential,
very low frequency/high consequence accidents — for the latter, see Ref. [120]) or sociopolitical (e.g. aversion,
opposition or proliferation). Some may be quantified in monetary terms and could be included in the CBA.
However, in most cases, such quantification has strong subjective elements, i.e. weighting factors may intervene
(see discussion in Ref. [118], p. 18). Therefore, in cases where non-monetary aspects are identified or anticipated as
a crucial part of the decision on which measures identified in an ALARP process should be implemented, a formal
MCDA may be best applied.
As mentioned in Ref. [72], if the risks (given by frequency multiplied by the consequences) are high, then the
demonstration of ALARP should be more rigorous than if the risk is low. Where risks are high, the consequences
should be weighted more than the frequency, and the ALARP demonstration should be accurate.
The technical assessment guide of the Office for Nuclear Regulation [72] provides specific guidance for
inspectors of nuclear installations on what they should expect of a licensee in meeting legal requirements to reduce
risks to ALARP. It suggests, on the basis of previous experience, a disproportion factor between costs and risk
reduction benefits of up to three for workers, whereas for risks to the public, the factor would depend on the level
of risk, suggesting a factor of about two for low risks and about ten for higher risks. These factors may be applied
in an analysis, unless other rationale are provided.
Owing to the uncertainties at various levels in the system under scrutiny and in the methods used for the
prediction of releases and associated consequences, it is suggested in Ref. [72] to base the judgement on the
appropriateness of the proportion factor on the robustness of the analysis. This factor should also be one of the
parameters to be varied in a sensitivity analysis
.
Considering that, usually, the scope of an environmental analysis includes also anticipated operational
occurrences, it is useful that the National Radiological Protection Board (NRPB) has produced guidance relating
to the dose saved resulting from routine exposure or minor incidents, i.e. stochastic effects, up to about 100 mSv.
The NRPB recommends that an increasing multiplier is used in ALARP demonstrations as the dose increases,
and also recommends the HSE Nuclear Safety Directorate to assess licensees’ submissions using this approach.
Reference [72] suggests that a factor (for costs outweighing risks) of less than ten in the vicinity of the intolerable
region is unlikely to be acceptable and, for hazards that can cause large consequences, the factor may need to be
larger still. This requirement may be understood as being even more pronounced for an NES under development,
especially at the early design stages for which the uncertainties may be predominant on the cost differences
calculated for risk reduction measures. The recommendation of Ref. [72], discussed above, to include the proportion
factor within the parameters to be varied in a sensitivity analysis is repeated here.
Example of application to a reprocessing facility
One of the rather few examples of the application of ALARP to a facility of a current NES [84] reports on the
Environment Agency’s re-examination of the Sellafield reprocessing plant’s radioactive discharge authorizations
from 2000 to 2002. This example would have the potential to also serve for assessing the ALARP concept of future
reprocessing plants. However, the information available in Ref. [84] is rather limited, and is therefore insufficient
for full understanding of the procedure used (e.g. missing information on risk levels before implementation of
ALARP, how the optimized actions were justified and on the costs involved).
Table 19 presents the doses to the critical groups caused by radioactive discharges from the Sellafield
reprocessing plant in 2001 [84].
Aerial effluent discharges | Liquid effluent discharges | ||
---|---|---|---|
Radionuiclide | Critical group dose (adult) (μSv) | Radionuclide | Critical group dose (seafood consumer) (μSv) |
Ar-41 | 31 | Tc-99 | 23 |
Sr-90 | 11 | C-14 | 5 |
Ru-106 | 3 | Cs-137 | 4 |
Cs-137 | 3 | Ru-106 | 2 |
Pu alpha | 2 | Sr-90 | 1 |
C-14 | 1 | Co-60 | 1 |
Kr-85 | 1 | Others | 1 |
I-129 | 1 | ||
Am-241 | 1 | Am-241* | 50 |
I-131 | 1 | Pu alpha* | 28 |
Others | 3 | Pu-241* | 4 |
Total | 58 | Total | 119 |
* - Historic contribution from discharges prior to the Site IonExchange Effluent Plant (SIXEP) and the Enhanced Actinide Removal Plant (EARP) have been introduced.
The strategy of the operator, British Nuclear Fuels (BNFL), for controlling radioactive discharges from the
Sellafield site consists of:
(a) Designing plants to minimize radioactive arisings, and subsequent discharges and disposals, where practicable
by:
- Employing processes that avoid the production of gaseous and liquid by-products, and that avoid solid waste generation (it is a general rule that because environmental impacts are greatest per unit activity for aerial releases, and are smallest for solid wastes, the design of the process reflects this hierarchy for the avoidance of generation of by-products).
- Proper design of abatement systems.
- Maximizing dispersion.
(b) Commissioning, operating and decommissioning plants, minimizing liquid and aerial discharges by means of:
- Prioritization in terms of dose impacts on the public. “To ensure resources are used in the most cost-effective manner, BNFL concentrates its ongoing research and development work on reducing those discharges which result in the highest doses or highest chemical toxicity, focusing effort on those areas in which there is the greatest potential for minimising environmental impact. For radioactive substances, it is generally the policy to use critical group dose as the key determining factor, though consideration is also given to collective doses” [84]. Table 19 shows that as of 2001 discharges, only a few radionuclides make almost all doses to the critical groups; this helps to identify where to intervene first in order to reduce risks to the population. Incidentally, the total critical group doses are within the BO–BL range of 0.02–1 mSv, as requested by the HSE.
- Increasing the magnitude of operations by decreasing the magnitude of wastes generated for eventual disposal.
- Addressing specific airborne emitted species: decreasing discharges of 41Ar, 14C and 129I.
- Addressing specific water-borne emitted species: abating 137Cs, 60Co, 239Pu and 99Tc.
(c) Developing new technologies.
(d) Implementing appropriate management systems.
Best available techiques
Concepts
According to the Convention for the Protection of the Marine Environment of the North-East Atlantic [13]: “The term ‘best available techniques’ means the latest stage of development (state of the art) of processes, of facilities or of methods of operation which indicate the practical suitability of a particular measure for limiting discharges, emissions and waste. In determining whether a set of processes, facilities and methods of operation constitute the best available techniques in general or individual cases, special consideration shall be
given to:
(a) comparable processes, facilities or methods of operation which have recently been successfully tried out;
(b) technological advances and changes in scientific knowledge and understanding;
(c) the economic feasibility of such techniques;
(d) time limits for installation in both new and existing plants;
(e) the nature and volume of the discharges and emissions concerned.”
Related to the application of BAT is the application of BEP, which is defined in the Convention [13] as “the application of the most appropriate combination of environmental control measures and strategies”. The Convention further states that [13]:
“In determining what combination of measures constitute best environmental practice, in general or individual cases, particular consideration should be given to:
(a) the environmental hazard of the product and its production, use and ultimate disposal;
(b) the substitution by less polluting activities or substances;
(c) the scale of use;
(d) the potential environmental benefit or penalty of substitute materials or activities;
(e) advances and changes in scientific knowledge and understanding;
(f) time limits for implementation;
(g) social and economic implications.”
In addition, BEP for a particular source will change with time “in the light of technological advances, economic and social factors, as well as changes in scientific knowledge and understanding” [13]. Finally [13]:
“If the reduction of inputs resulting from the use of best environmental practice does not lead to environmentally acceptable results, additional measures have to be applied and best environmental practice redefined.”
Regardless of the approach that is used for determining the optimal option, it should be recognized that
judgements are required about the relative significance of the factors involved, which includes dialogue between
the regulatory body and the operator, as well as other interested parties, particularly those who might be affected by
the situation, facility or planned activity (e.g. local community representatives).
Formal decision aiding techniques may be used in the optimization process, especially for nuclear fuel cycle
facilities. However, when the doses to the representative person are assessed to be very low (e.g. of the order of
10 μSv/a or less), a formal analysis of the optimization of protection is generally not necessary. The magnitude
of exposure, the number of exposed individuals and the likelihood of exposure should be assessed for each of the
options being considered. For complex facilities, elements of the radiological assessments should be made available
at an early stage, and the development of the assessment may be an iterative process involving discussions between
the operator, the regulatory body and other interested parties. The assessment should be refined as the development
of the facility moves towards the stage at which an application for a licence for the planned facility is to be made
(e.g. a licence to operate).
Application of best available techniques in environmental impact assessment
BAT assessment is typically undertaken when making decisions regarding the development and
implementation of appropriate discharge standards to ensure environmental protection. This can include situations
when standards for the discharge of radioactive material are being developed, for planning the management and
disposal of radioactive waste, when authorizations are undergoing a significant amendment or when facilities are in
a sensitive environment.
An example is provided here for the Gunnar Mine Site, which is an abandoned uranium mine and mill site
in northern Saskatchewan, Canada. The site was operated between the late 1950s and early 1960s under a less
stringent regulatory regime than today. Uranium ore was initially mined from an open pit from 1955 to 1961, and
later, from an underground operation between 1957 and 1963. The Gunnar Mine Site officially closed in 1964, with
little or no remediation of its facilities.
A stepwise decision tree approach was developed and applied to identify all potentially feasible remedial
options, as part of the EIA process, and to map out key decision points during the licensing phase of the project,
when final remedial options were selected from the list of options. Although the example provides an illustration of
the approach for the remediation of the Gunnar Mine Site, the same approach can be used for optimization of the
options intended for environmental protection.
A number of end points (or desired outcomes of the remediation efforts) were identified for the site, as
follows:
- The site does not pose unreasonable public health or environmental risks.
- Flora and fauna adjacent to the site are not significantly affected by contaminants.
- Traditional use of resources adjacent to the site can be safely conducted.
- The desire is to have the site managed through an institutional controls programme for long term care and maintenance.
Screening framework for evaluation of remedial options
In conducting the alternatives assessment, all available options were identified and evaluated, including all
aspects of each mine waste disposal alternative throughout the project life cycle, and all aspects of the project
(direct or indirect) that may contribute to the predicted impacts associated with each potential option [124, 125].
The assessment considered the predicted quality and quantity of releases to the environment, and the corresponding
predicted effects on the environment. The assessment of alternatives also considered the full cost of each remedial
option throughout the project life cycle and its associated benefits, as an independent step from the technical review
of options.
Assessment of the predicted quality and quantity of releases to the environment, and corresponding effects to
the environment, are addressed through characterization of contaminant concentrations and key contaminant flow
pathways, and subsequently inputting data into the human health and ecological risk assessment (HHERA).
Evaluation of remedial options was conducted using a multitiered assessment approach based on
environmental and technical CR, utilizing the framework for assessment of alternatives proposed in Ref. [124], and
which is presented in Table 20.
Step | Definition | Criteria | Analysis | Deliverable |
---|---|---|---|---|
1 | Identify candidate alternatives | Threshold criteria | Mine waste disposal technologies
Mine waste disposal storage options Mine waste disposal sites |
Summary table
Location maps |
2 | Prescreening assessment | Prescreening criteria | Prescreening evaluation | Summary table |
3 | Alternative characterization | Characterization criteria (accounts) | Environmental criteria
Technical criteria Project economic criteria Socioeconomic criteria |
Summary tables |
4 | Multiple accounts ledger | Evaluation criteria (subaccounts)
Measurement criteria (indicators) |
Qualitative value scales | Summary tables |
5 | Value based decision process | Scoring
Weighting |
Quantitative analysis | Summary tables |
6 | Sensitivity analysis | Reconsider weighting | Quantitative analyses | Summary tables |
7 | Document results | Final report |
The summary of the major steps of the assessment provided in Ref. [125] specifies that these steps include:
- Development of a list of all possible candidate remedial options, with the input from stakeholders and without prior judgements;
- Screening of options to reduce the number and to provide assurance that any of the remaining options could prove to be the preferred option;
- Characterization of the remaining alternatives to ensure proper consideration of every aspect and nuance and the comparison of alternatives in a clear and concise format, to ensure complete transparency of the decision process;
- Identification of evaluation criteria that are linked to an effect and that easily differentiate the alternatives;
- Inclusion of quantitative value judgement in the decision process by scoring and weighting all evaluation criteria under the environmental, technical, economic and socioeconomic characteristics of each alternative;
- An assessment of the sensitivity of the decision making process.
An assessment of candidate Gunnar remedial options was conducted in general accordance with the framework for MCDA provided by Ref. [124], which is provided as a case study.
Remedial options analysis
A description of the key steps in remedial options analysis (see Table 23) was developed by Environment
Canada in 2011 [124]. This approach has been applied to evaluate unfiltered candidate options for remediation at
the Gunnar Mine Site.
Step 1: Identification of candidate alternatives
A first step in the assessment of remedial options is to develop a list of all possible candidate remedial
options, with input from stakeholders and without prejudgement, i.e. to generate an ‘unfiltered’ list of remedial
options [124, 125]. In doing so, a basic set of ‘threshold criteria’ (or ‘exclusion criteria’) may be established that
can then be used in the prescreening of unfiltered options, as part of step 2 of the remedial options analysis.
For the purposes of options assessment, candidate alternatives were identified using a two phased approach:
compilation of an unfiltered list of remedial options and then the evaluation of this list. The unfiltered list of
remedial options that was generated during the first phase was distributed for review and input to responsible
authorities, including provincial and federal regulatory and project partner stakeholder groups, in the second phase.
The unfiltered remediation alternatives were generated to address each of the following Gunnar Site components,
which were identified in the Gunnar EIA as the major ‘aspects’ of the site remediation:
- Waste disposal (i.e. disposal of waste generated by the demolition of former site infrastructure);
- Waste rock deposit;
- Open pit;
- Hydrocarbon contaminated soil;
- Waste rock seepage;
- Groundwater seepage;
- Gunnar main tailings (including tailings downstream of the dyke at the eastern boundary of the Gunnar main tailings deposit);
- Gunnar central tailings;
- Langley Bay/Back Bay (including tailings and aquatic receiving environment).
The candidate alternatives that were generated through this exercise constituted a broad list of waste disposal
technologies, storage options and disposal locations, both on-site and off-site.
Step 2: Prescreening assessment
Following identification of the candidate remediation alternatives (as part of step 1 above), a prescreening
assessment of the unfiltered list was conducted, based on threshold criteria (or exclusion criteria). The objective of
the prescreening was to optimize the decision making process, with focus on an appropriate and manageable set of
sufficiently detailed alternatives, as opposed to evaluating options that have obvious deficiencies [124].
Once the generic alternatives had been identified, based on reason, practicality and precedence, any ‘fatal
flaw’ for each alternative was identified via preliminary screening. The screening of options was performed against
threshold/exclusion criteria specific to the project objectives/desired end points. These criteria were established so
as to generate a simple ‘yes’ or ‘no’ answer, dictating which alternatives were to be carried through to more detailed
analysis. The established threshold (or exclusion) criteria were as follows:
- Does the alternative allow for compliance with a total external gamma dose criteria (mean value of ≤ 1 μSv/habove the background at a 1 m height, averaged over a 100 m × 100 m area and maximum value of 2.5 μSv/habove the background at a 1 m height, based on close out criteria for Cluff Lake, a uranium mine/mill sitein northern Saskatchewan further along in the decommissioning/remediation process than Gunnar [126])(Gunnar background = 0.7 μSv/h)? (Answer of ‘no’ indicates a fatal flaw.)
- Does the alternative allow for long term compliance with site specific/Saskatchewan Environment/Canadian Council of Ministers of the Environment guidelines/objectives/standards for soil and surface water quality? (Answer of ‘no’ indicates a fatal flaw.)
- Does the alternative allow for compliance with pertinent provincial/federal legislation? (Answer of ‘no’ indicates a fatal flaw.)
- Is the alternative a proven technology/has it been completed elsewhere with successful results? (Answer of ‘no’ indicates a fatal flaw.)
- Does the alternative improve the level of risk at the site? (Answer of ‘no’ indicates a fatal flaw due to an increase in risk.)
- Is there a potential risk of catastrophic failure? (Answer of ‘yes’ indicates a fatal flaw.)
- For waste disposal, is the proposed disposal site sufficiently large to accommodate waste? (Answer of ‘no’ indicates a fatal flaw.)
Each alternative receiving a positive evaluation (i.e. with no fatal flaws identified) was carried through a
multiple accounts analysis (as demonstrated in Table 20), where the impacts from each alternative were assessed
through a transparent scoring and weighting process.
Step 3: Characterization of alternatives
Once the prescreening assessment of candidate remedial alternatives had been completed, the remaining
options required characterization. During options characterization, the options for each major site element were
evaluated by a multidisciplinary project team consisting of individuals with expertise in the natural sciences,
contaminant dynamics and engineering. Only potentially feasible alternatives were carried through to this stage
of analysis. Feasible alternatives included those that could represent implementable remedial options, with no
fatal flaws being identified during prescreening with respect to project objectives and end points. For clarity and
differentiation from prescreening of remedial ‘alternatives’, potentially feasible alternatives that were carried
through to detailed analysis were renamed as ‘options’ (as opposed to alternatives).
The outcomes of the evaluations of HHERA were derived in the context of the following broad evaluation
criteria, which were developed for the site as part of the Gunnar EIA:
- Human health risks;
- Terrestrial wildlife and vegetation;
- Aquatic life and vegetation.
Within each of the above broad criteria, more detailed evaluation criteria (or ‘subaccounts’) were established,
as described under step 4 below.
The focus of the evaluation of options with respect to HHERA was centred around potential option related
chemical and radiological impacts to members of the public and the environment. In addition, physical impacts
to terrestrial and aquatic life and vegetation were considered as part of the ecological risk assessment related
evaluation criteria. As the Gunnar Mine Site has been secured and is not open to the public without orientation, and
work activities are being planned and implemented to protect people, physical impacts to humans were considered
as part of the evaluation of constructability.
The constructability evaluation was focused on the following two evaluation criteria:
- Potential risks to humans (i.e. workers) and the environment during the execution of the remediation (as part of the ‘active remediation phase’);
- Constructability/feasibility/efficacy of a given remedial option from a ‘practicability of execution’ perspective.
Following evaluation of remedial options with respect to HHERA and constructability, further screening was
conducted to identify the potentially acceptable options based on public preference, gained through engagement
of local communities and leaders, both through providing residents with information on the project, and receiving
feedback and advice from communities. This was accomplished through community meetings, surveys and
other forums, which provided important insight with respect to public preferences regarding the planning and
implementation of the project, and which were considered in the evaluation of the Gunnar remedial options.
Step 4: Multiple accounts ledger
Following identification and characterization of alternatives in steps 1–3, it was necessary to then evaluate the
options that were identified as potentially feasible, by identifying elements that could be used to differentiate them.
This was accomplished through the development of a multiple accounts ledger, which consisted of the following
two elements: subaccounts (also called evaluation criteria) and indicators (also called measurement criteria).
Subaccounts (or evaluation criteria) were developed using the characterization criteria that had been selected
during step 3. Unlike the characterization criteria, which were factual criteria that had been assigned with no a
priori judgement being made regarding any of the alternatives under consideration, the evaluation criteria were
developed with consideration of the material impact (benefit or loss) associated with each of the options under
evaluation.
Evaluation criteria with respect to the Gunnar remediation project were, therefore, set in the context of three
broad categories (or ‘accounts’): HHERA, constructability and public preference, as described above.
Within the above evaluation criteria, a number of qualitative and quantitative measurement criteria were
established, which were then scored to identify potentially feasible remedial options.
The following measurement criteria were identified with respect to potential worker and ecological health
risks during remediation:
- Physical hazards (safety risks);
- Worker health risks via exposure to non-radiological and radiological contaminants (soil or dust inhalation, dermal contact), ambient radon exposure and external radiation exposure;
- Risks to terrestrial wildlife generated;
- Risks to aquatic life generated.
The following measurement criteria were identified with respect to constructability, feasibility and efficacy during remediation:
- Estimated construction duration;
- Cost;
- Ability to mitigate pertinent exposure pathways;
- Regulatory acceptance;
- Long term waste generation;
- Requirement for control, monitoring and/or maintenance (i.e. institutional control).
The possible Gunnar remedial options were evaluated in the context of a single integrated measurement
criterion that has been identified as ‘public preference’, which was scored as described in further detail below.
Step 5: Value based decision process
Once a multiple accounts ledger had been created, with criteria to facilitate the evaluation and measurement
of possible remedial options, it was necessary to score and weight all the indicators, subaccounts and accounts,
such that merit ratings for each option could be quantitatively determined. In doing so, the following processes
were undertaken:
- Scoring;
- Weighting;
- Quantitative analysis.
Scoring of the possible Gunnar remedial options from the perspective of HHERA in the long term was conducted using both quantitative and qualitative measurement criteria. Quantitative criteria were based on the concentrations of contaminants released to the environment in the long term for a given option relative to environmental quality guidelines, whereas qualitative criteria provided a relative comparison of remedial options in cases for which no quantitative guidelines existed against which measurement criteria could be compared. On this basis, the following scoring criteria were developed:
- A value of 0 indicates exceedance of the guideline by 100-fold or greater/physical risk that exceeds the baseline risk; this option performs very poorly against listed criteria.
- A value of 1 indicates exceedance of the guideline by 10- to 100-fold/physical risk that is similar to the baseline risk; this option is the same or has a very small improvement compared with the existing situation.
- A value of 2 indicates exceedance of the guideline by 1- to 10-fold/physical risk that is lower than the baseline risk; this option has a moderate improvement compared with the existing situation.
- A value of 3 indicates not applicable; this option has no exceedance of guideline values or a large improvement compared with the existing situation/best performing option.
To differentiate the performance of the options versus the prescribed criteria, numerical rankings were assigned as a quantitative indicator of impact. The following measurement scheme was used with respect to constructability:
- A value of 0 indicates the option performs very poorly against listed criteria.
- A value of 1 indicates the same or a very small improvement compared with the existing situation.
- A value of 2 indicates moderate improvement compared with the existing situation.
- A value of 3 indicates not applicable/large improvement compared with the existing situation/best performing option.
In terms of options that were ‘do nothing’ or very near to do nothing, many of the criteria in the technical
analysis were evaluated as ‘not applicable’. These not applicable designations were given a ranking of 3, to indicate
a high ranking in terms of subaccounts, such as duration or cost, in comparison with options for which some action
is taken.
Scoring of each remedial option with respect to public preference was undertaken using an integrated approach
to incorporate information that had been received from community members and leaders through a diversity of
consultation approaches. The outcomes of such approaches, which included community meetings, flyers, surveys
and many others, were used to inform the scoring in the context of the following scoring criteria:
- A value of 0 indicates this option is unacceptable or that local communities are opposed to the option.
- A value of 1 indicates local communities would consider it as a possible option, but with reservations.
- A value of 2 indicates local communities would consider it as a possible option.
- A value of 3 indicates local communities would consider it as a preferred option.
It should be noted that in assigning scores of 1 versus 2 above, values were set to distinguish options that
were considered possible by local communities, in one case, with reservations, and in the second case, with few
to no reservations. For example, a score of 1 was assigned for options involving transport of hazardous materials
across Lake Athabasca. In such scenarios, it was necessary for activities to be conducted in close consultation with
communities owing to the value that northern communities place on water quality and the concerns of catastrophic
releases of hazardous materials to Lake Athabasca. Despite these reservations, it was possible to transport hazardous
materials across the winter ice road, working closely with local communities.
As part of the analysis, weighting factors were applied to the raw scores that had been generated during the
evaluation of possible Gunnar remedial options. In this way, it was possible to assign a relative importance of a
given measurement criterion compared to others.
As a preliminary step, weighting factors were assigned to each of the three accounts (HHERA, constructability
and public preference), as follows:
- A value of 40% for HHERA;
- A value of 40% for constructability;
- A value 20% for public preference.
Within each of the above three accounts, individual measurement criteria were also weighted and justified to
reflect their relative importance.
Once scoring and weighting of each possible remedial option was completed, it was necessary to conduct a
quantitative analysis of the information. This involved compiling the indicator value S (‘score’) for each indicator
(or measurement criterion), along with the weighting factor W, and calculating the product of these to generate a
combined indicator merit score (S·W) for each possible remedial option [124].
Based on these data, it was possible to take the sum of the indicator merit scores for each evaluation criterion
(or subaccount) to generate a subaccount merit score for each, i.e. Σ{S·W}. Within a given subaccount (or evaluation
criterion), the resultant subaccount merit scores could then be directly compared across possible remedial options.
To facilitate further comparison of each subaccount merit score against values for other subaccounts, the
scores were normalized to the same point scale used to score each indicator value. In doing so, the subaccount
merit score was divided by the sum of the weightings (Σ W) to yield a subaccount merit rating [124]:
The same procedure can be undertaken to weight and normalize scoring for accounts (in this case, HHERA,
constructability and public preference) to generate account merit scores (Σ{Rs·W}) and account merit ratings
(Σ{Rs·W}/ΣW) for each remedial option.
As a final step, option merit scores (Σ{Ra·W}) and option merit ratings (Σ{Ra·W}/ΣW) can be determined for
each possible Gunnar remedial option.
It should be noted that even with the multiple layers of analysis, it is possible for certain options to score
identically, against all criteria, for a certain aspect of the site. In the event of such scoring/ranking, the performance
of the option needs to be measured against the total available score, as described below.
Step 6: Sensitivity analysis
Any decision making process will be inherently subject to some degree of bias and subjectivity. Therefore,
it is important that a framework is followed to ensure that any decisions arising from the options screening and
selection process are transparent and reproducible by external reviewers [124]. With this in mind, the options
analysis was completed for the Gunnar Mine Site in multiple tables, rating the potential remedial options separately
with respect to performance against: (i) HHERA, (ii) constructability and (iii) public preference, to allow for such
transparency.
A sensitivity analysis can involve a very large number of permutations and combinations, which in itself can
impose bias and subjectivity. Therefore, for the purposes of the Gunnar evaluation, the sensitivity analysis was
kept simple, with the ultimate goal of evaluating the impact of the respective indicator weights (W) on the selection
of potentially feasible remedial options, based on the initial ranking (i.e. assignment of a value of 0–3 for each
measurement criterion and remedial option combination). As such, the analysis was conducted by simply setting all
weightings to a value of 1, effectively removing the value bias between one measurement criterion and another. In
this case, remedial options achieving relatively higher sums of indicator values measured against the measurement
criterion values may be more feasible for a given site aspect than those with lower indicator values. A sensitivity
analysis was conducted to evaluate this.
Summary of the study results
An assessment of candidate remedial options was conducted in general accordance with the framework for
MCDA provided by Ref. [124]. The analysis consisted of multiple stages of analysis, as demonstrated in Table 23.
Based on this approach, a comprehensive, unfiltered list of possible remedial options for the Gunnar Mine Site was
evaluated and scored to identify potentially feasible remedial options.
For the purposes of this analysis, a potentially preferable option was identified as the option for which the
highest option merit rating was determined. Owing to the inherent complexity of the Gunnar remediation project,
it was necessary to subdivide remedial options into a number of interconnected site aspects (e.g. submerged and
unsubmerged unconfined tailings, waste rock piles, open pit, etc.), which were individually scored as separate
matrices, with respect to HHERA and public preference.
Based on this analysis, potentially preferable remedial options were identified to address each Gunnar Site
aspect; however, due to the lack of historical records and monitoring data, it was not possible to identify a single,
‘preferred’ remedial option. Instead, potential remedial options could be subdivided into three categories [127]:
(a) Options that are relatively straightforward to implement, with little uncertainty, e.g. covering unconfined
tailings, water diversions, removal of contaminated debris from waste rock piles and addressing physical
hazards associated with unstable slopes on the waste rock piles.
(b) Options that require additional monitoring data and follow-up assessment prior to selection of a final
remediation approach, e.g. further surface water and hydrogeological monitoring to determine contaminant
transport pathways between sources and receiving environments.
(c) Options that are dependent upon the selection of remedial approaches for other site aspects that have to be
determined before the first one can proceed, e.g. decisions on the pit cannot be determined until it has been
decided if the waste rock will be placed in the pit or covered and left in place.
Appendix IV
Concept of collective dose
The concept of collective dose was found to be very useful for comparative analysis of radioactive emissions
from nuclear facilities and systems.
Introduction
Interpretation of the collective dose has been discussed by the ICRP, which, in its recommendations [128],
believes that the use of the collective dose concept is closely connected with formal CBA. The product of the
average dose to an individual and the number of individuals in a group (i.e. how collective dose has been calculated
in the past) is a legalized quantitative value, but it is of a limited application, as it combines redundant information.
For decision making, the necessary information has to be presented as a matrix that indicates the number of
individuals exposed to a specific dose and the date it was received. This matrix needs to be considered as an
auxiliary decision making tool allowing the significance evaluation of individual matrix elements. The matrix
approach leads to a more correct estimation of consequences (risks) of irradiation. The ICRP believes that this
will avoid misinterpretation of the collective dose, which has resulted in serious errors in the prediction of lethal
outcomes. A justification of these ICRP recommendations can be found in Ref. [128].
Collective doses are generally not used by regulators; instead, regulators usually limit the emission of single
radionuclides or classes of radionuclides represented by equivalent isotopes on the basis of doses to the critical
group. However, collective doses normalized per unit of electricity were used in the ExternE project for the
calculation of external costs for comparing different power technologies [110, 111, 119]. Annual collective doses
have also been used for comparison of different options of nuclear cycles in Ref. [116]. Recommendations on how
collective doses should be calculated in particular cases can be found in Refs [23, 129, 130].
Use of collective doses also presents some disadvantages, e.g. the possibly prevailing importance given to
very low doses occurring over very long time periods (applying the linear dose effect relationship without cut-offs),
the dependency on key modelling assumptions that are not easily controllable such as population distributions
(in place and time) and the application, or not, of discounting.
Simplified determination of collective dose
The IAEA generic models (see also Appendix II) include a simplified method to determine collective doses.
In section 7 of Ref. [23], tables contain collective effective dose commitments per unit activity (man Sv/Bq)
of radionuclides discharged to the atmosphere, to marine water and freshwater bodies. All contributions from
individual radionuclide species and pathways need to be summed.
The simplified conversion of release rates to dose factors has been derived using the results of two approaches:
one based on a simple method using generic parameters [98] and the other one based on complex modelling [131],
which have been developed by the NRPB, in the United Kingdom. Reference [23] suggests using simplified models
with caution, noting that they can only provide order of magnitude estimates, and that collective doses:
“...should be used only as part of a screening or generic assessment procedure, for example to ensure compliance with dose limiting criteria or as input to an optimization exercise to compare options as part of an intuitive, semi-quantitative analysis. They should not be used for more rigorous optimization analyses, such as cost–benefit analyses, nor for other purposes.”
Reference [23] further states that “the site specific discharge conditions and the actual critical group location
be taken into account if the predicted doses exceed a reference level of around 10% of the dose constraint.”
Collective doses were estimated for most radionuclides only in local and regional zones, which may extend
from the point of release to distances varying from about a hundred kilometres to several thousand kilometres [23].
Four nuclides were considered for global analysis because of their relatively long radioactive half-lives or a high
environmental mobility: 14C, 3H, 129I and 85Kr; other long lived radionuclides, such as 237Np or 99Tc, may also
become globally dispersed following discharge, but they have not yet been accurately addressed. Therefore,
an analyst may need to consider these (and others), depending on the technical characteristics and expected
performance of the NES.
Collective doses can be used for the purposes of optimization of radiation protection measures. WS-G-2.3 [33]
recommends using the estimations of collective dose arising from discharges to avoid spending resources to assess
options for reducing discharges in disproportion to the likely improvement in radiological protection. The collective
dose from discharges, which can be estimated using Ref. [23], should be added to an estimate of the relevant
collective dose from occupational exposure to provide an estimate of the total collective dose. If the result is less
than about 1 man Sv/a, an extensive formal optimization study most probably will not be needed [132]. If the value
of the collective dose is greater than about 1 man Sv/a, a formal study by the designer (technology developer) is
required, with the use of decision aiding techniques such as CBA and multicriteria methods.