Enhancing benefits of nuclear energy technology innovation through cooperation among countries
Contents
- 1 Summary
- 2 Introduction
- 3 Main issues for sustainability enhancement of an NES and possible solutions – the SYNERGIES storylines
- 4 The SYNERGIES case studies
- 5 Cooperative solutions on regional/global levels
- 6 Technical synergies among reactor technologies
- 7 Drivers for and impediment to synergistic approaches
- 8 Near and medium-term actions towards enhanced nuclear energy sustainability
- 9 Insights on the synergistic approach and its implementation
- 10 Conclusion
- 11 References
Summary
The International Project on Innovative Nuclear Reactors and Fuel Cycles (INPRO) was established in 2000 to help ensure that nuclear energy is available to contribute to meeting the energy needs of the 21st century in a sustainable manner. INPRO focuses on key issues of global sustainability of nuclear energy, with the aim of assisting in the development of long term nuclear energy strategies.
This brochure presents the main findings of the INPRO collaborative project ‘Synergistic Nuclear Energy Regional Group Interactions Evaluated for Sustainability’ (SYNERGIES). It was undertaken within the INPRO area on ‘global scenarios’, which analyses regional and global nuclear energy scenarios to achieve a global vision of how nuclear energy could be sustainable within the present century.
The final report of the SYNERGIES project was published in the IAEA Nuclear Energy Series in 2018. [1]
The project was implemented in 2012–2015 by experts from 23 IAEA Member States and one international organization. In conducting case studies, the participating countries addressed two main questions:
- Can a globally sustainable nuclear energy deployment be realised?
- Which technologies and forms of international collaboration would be needed to provide the path towards worldwide sustainable deployment of nuclear energy?
The case studies from these widely diverse countries modelled and examined the ability of a synergistic approach to enhance sustainability of a nuclear energy system (NES) by improving (i) efficiency and competitiveness; (ii) utilization of resources and waste; (iii) proliferation resistance; (iv) safety and security; and (v) social and public acceptance.
The project concluded that some technical synergies among reactor technologies of different types and their fuel cycles were already implemented in a few countries, thus demonstrating their practical value. This could be extended considerably to solve issues related to enhancing fissile materials utilization, waste management, and improving economic competitiveness and proliferation resistance.
Using a synergistic approach, the project also examined the collaboration among nuclear energy technology holders and users. The results of the case studies provided a better understanding of the drivers and impediments of different forms of partnerships in developing national nuclear power programmes.
The project identified several forms of collaboration among countries, such as collaboration (nuclear trade) on the nuclear fuel cycle front end and back end; support by technology holders to national R&D and deployment programmes of technology users; steady regional collaboration; and joint development of instruments for the simulation of collaborative scenarios, which could help enhance regional and global sustainability of nuclear energy.
Introduction
In 1987, the United Nations World Commission on Environment and Development Report [2] (often known as the Brundtland Commission Report), defined sustainable development as “development that meets the needs of the present without compromising the ability of future generations to meet their own needs”. The Brundtland Commission Report inter alia presented comments on nuclear energy in Chapter 7, Section III. According to them, in the area of nuclear energy, the focus of sustainability and sustainable development is on solving certain well-known problems (referred to as “key issues”) of institutional and technological significance. In line with it, seven key issues were identified and discussed in the Brundtland Commission Report:
- Proliferation risks;
- Economics;
- Health and environment risks;
- Nuclear accident risks;
- Radioactive waste disposal;
- Sufficiency of national and international institutions (with particular emphasis on intergenerational and transnational responsibilities);
- Public acceptability.
Since then, several significant events related to sustainable development have taken place; however, none of them changed the definition given in the Brundtland Commission Report[3][4].
In particular, the United Nations Conference on Sustainable Development (Rio+20), held in 2012, resulted in an outcome document, “The Future We Want”[5], which contains practical measures for implementing sustainable development. At Rio+20, Member States committed to develop a set of Sustainable Development Goals (SDGs) building on the United Nations Millennium Development Goals and converging with the post-2015 development agenda.
In 2015, a new programme was started to develop the universal, integrated and transformative 2030 Agenda for Sustainable Development. This agenda was launched at a summit in September 2015, and the United Nations General Assembly formally adopted the universal, integrated and transformative 2030 Agenda for Sustainable Development, along with a set of 17 Sustainable Development Goals (SDGs) and 169 associated targets[6].
There are two principle SDGs with which NESs directly connect: affordable and clean energy (SDG 7) and climate change (SDG 13). Enhancing nuclear energy sustainability essentially translates into removal of many public acceptance concerns and, eventually, in an increase of the nuclear share in global energy production, with associated positive effect on climate change mitigation.
The activities of INPRO are centred on the key concepts of global nuclear energy sustainability and the development of long-range nuclear energy strategies, so that nuclear energy is and remains available and affordable to meet national energy needs. In cooperation with IAEA Member States, INPRO has defined the requirements for a sustainable NES consistent with the United Nations concept of sustainable development.
To address the important specific issues relevant to the development and deployment of NES, INPRO has established a methodology for assessing the sustainability of NES[7][8][9][10][11]. The INPRO methodology groups the United Nations sustainable development concept into seven subject areas important for nuclear energy: economics, waste management, infrastructure, proliferation resistance, physical protection, environment and safety.
About 10,500 tons is being added annually to the global stock of 270,000 tons of the accumulated spent nuclear fuel awaiting decisions by the national governments in countries using nuclear power. The amount of spent nuclear fuel will continue to grow with an expected increase in nuclear power unless safe, secure and sustainable solutions are implemented. This is one example of the main challenges for sustainability of nuclear energy systems, others being concerns about economic competitiveness, safety, proliferation resistance and security of nuclear energy. Despite considerable development of nuclear technologies, finding competitive and environmentally benign, safe and secure solutions that are socially and politically acceptable remains a tall order.
One of the activities of INPRO in the area of global scenarios” implements scenario studies to understand key issues and opportunities for a transition to future nuclear energy systems (NES) with enhanced sustainability. Activities are aimed at development and exploration of long-term scenarios to identify technological options, economic performance, infrastructural and collaborative arrangements of the future NES that would enhance regional and global sustainability of nuclear power.
Several collaborative projects were and are being implemented within the “Global scenarios” area on development and application of the analytical tools for modelling NES scenarios to identify opportunities for joint actions on technological innovations and cooperation among countries for enhancing sustainability of NES. One of such collaborative projects was titled ‘Synergistic Nuclear Energy Regional Group Interactions Evaluated for Sustainability’ (SYNERGIES)[1]. It was a follow-up of the collaborative project ‘Global Architecture of Innovative Nuclear Energy Systems with Thermal and Fast Reactors and a Closed Fuel Cycle’ (GAINS)[3], which developed the analytical framework for analysis and assessment of transition scenarios to sustainable nuclear energy systems[12]. SYNERGIES project was established in response to a strong interest expressed by INPRO members to further develop and apply the synergistic approach of GAINS, now more on a regional rather than global level, as a method for evaluating the means of technological innovations and cooperation among countries (nuclear trade) for enhancing the sustainability of nuclear energy. Its main objective was to identify and evaluate mutually beneficial forms of collaboration, and the driving forces and possible impediments involved in achieving regionally and globally sustainable NES built on a synergistic combination of (i) mature and innovative nuclear energy technologies, and (ii) different forms of collaboration (nuclear trade) among countries. Synergies within the context of nuclear energy are those actions that a country or a group of countries may undertake to facilitate (i.e., enable, accelerate and optimize) the deployment of the nuclear energy systems with enhanced sustainability.
The project was implemented in 2012-2016, under the IAEA auspices, by experts from Algeria, Argentina, Armenia, Belgium, Bulgaria, Canada, China, France, India, Indonesia, Israel, Italy, Japan, Republic of Korea, Malaysia, OECD-NEA, Pakistan, Romania, Poland, Russian Federation, Spain, UK, Ukraine, USA, and Viet Nam. Diverse membership of the participating countries with different preferences and competence in nuclear power development and international collaboration was a major strength of the project.
This page presents a short summary of the main conclusions of the SYNERGIES project.
Main issues for sustainability enhancement of an NES and possible solutions – the SYNERGIES storylines
The concept of a sustainable NES was introduced in INPRO to enhance the ability of nuclear energy to contribute to meeting energy needs of the 21st century in accordance with the principles and goals of the United Nations’ Agenda for sustainable development[13][14]. Two methodological approaches for sustainability assessment were developed in INPRO: “Nuclear Energy System Assessment (NESA)[7][8][9][10][11], a methodology for a holistic assessment an NES, and a framework for analysis and assessment of transition scenarios to sustainable nuclear energy systems, the GAINS framework[13].
The GAINS approach to defining sustainability metrics builds upon the INPRO methodology for NES assessment but in most cases, does not duplicate it. It is narrower and focuses on the areas that are important for scenario analysis (i.e. can be assessed through material flow analysis and associated economic analysis). The major areas considered in GAINS are resource availability and production of waste, the associated power capacity curves for nuclear reactors involved, radioactivity and radiotoxicity of waste, demand in fuel cycle services and costs and the required investments. Other important areas such as safety and physical protection are not covered under the GAINS metrics but are assumed to be compliant with other assessments based on the INPRO methodology.
The GAINS notion of sustainability and the GAINS metrics[13] were used and elaborated in the studies carried out within the SYNERGIES project. The objective was to examine the scenarios moving both technology holders and technology users towards sustainable nuclear energy solutions in terms of sufficient resources and minimum waste, timely achievement of targeted capacity evolution curves, minimization of long lived radiotoxicity, amplifying the benefits of innovative technologies that are costly to develop to bring them to a wide range of users in an affordable way through mutually beneficial cooperation with technology holder countries in the nuclear fuel cycle.
In terms of the scope of the SYNERGIES project (focused on the material flow and economic analyses), the major long-term sustainability enhancement issues addressed were as follows:
- (a) Progressive accumulation of spent nuclear fuel that creates a burden for future generations;
- (b) Non-effective use of natural fissile resources that in the future might create problems related to fissile resource non-availability;
- (c) Presence of direct use materials (plutonium) in spent nuclear fuel, first in irradiated form, and, in several hundreds of years, already in a form that might be rated as unirradiated and that might create long lasting (hundreds of thousands of years) proliferation resistance and security concerns in the case of direct disposal of spent nuclear fuel in non-nuclear-weapon States;
- (d) Huge investments required to develop and deploy innovative technologies for nuclear power, making such innovative options unaffordable for many current and potential users of nuclear technology;
- (e) Risks related to global spread of sensitive technologies of uranium enrichment and spent fuel reprocessing, addressing the consequences of which would be a huge burden for future generations.
It is well recognized that not all the countries using or planning to use nuclear energy can address indigenously all the sustainability issues listed above. Even if technically possible for some of such countries, it would not be economic to solve all the sustainability issues in isolation. The majority of these countries would thus have or opt to rely on imported ‘off the shelf’ nuclear energy technologies and supply of nuclear fuel and other services and would increasingly demand regional or/and international cooperation among countries. Can a global sustainable nuclear energy deployment be realised, and which technologies and what forms of international collaboration would be needed to provide the path towards worldwide sustainable nuclear deployment? This was the main question addressed by the SYNERGIES collaborative project, through case studies by the participating countries. These studies were designed around a storyline to encompass a full spectrum of alternatives, as shown in Fig. 1.
The architecture of this storyline is built on the existing, evolutionary and innovative technological options for enhancing nuclear energy sustainability. The technology related options were structured along generic fuel cycle options, with generic reactor options linked to fuel cycle options. The reason for this is that the generic reactor technologies may be common for several generic fuel cycle options, while the generic fuel cycle options are limited in number and well known. The details on nuclear reactors associated with the defined fuel cycle options are provided in Appendix V of Ref.[1].
With respect to fuel cycle technology, the following options have been defined:
Option A. Once through nuclear fuel cycle
This is currently the most widespread, although not the only option realized in the majority of countries using nuclear energy. The reactors currently operated in a once through fuel cycle include multiple light water reactors (LWR) including those with a graphite moderator, gas cooled reactors, heavy water reactors (HWR) and also some additional reactor types.
Option B. Recycle of spent fuel with only physical processing
This option provides for a single recycle of spent nuclear fuel from reactors of a particular type in nuclear reactors of another type, with no chemical reprocessing applied. It could help to a small extent save natural uranium resources and reduce spent nuclear fuel volume for final disposal, while avoiding the use of proliferation sensitive chemical reprocessing technology. The concluded R&D on this technology in application to LWR spent nuclear fuel single recycle in HWR — the direct use of spent PWR fuel in CANDU reactors (DUPIC) process — is deemed sufficient for practical implementation. However, this had not taken place so far.
Option C. Limited recycling of spent fuel
This enhancement option is a step in improving resource utilization and reducing the waste burden. Limited recycling reduces spent nuclear fuel volumes, slightly improves resource utilization and keeps fertile fuel resources more accessible for later options of sustainability enhancement, thus offering some flexibility for long term management of nuclear materials. The achieved effects are similar in magnitude, albeit somewhat larger than in Option B described above.
This option requires the development and deployment of proliferation sensitive commercial spent fuel (chemical) reprocessing and the fabrication of fuel from previously irradiated materials. Some Member States have already deployed these technologies and are successfully operating them on a commercial scale for several decades, providing spent nuclear fuel take back services with the return of ‘ultimate’ waste — vitrified mixture of fission products and minor actinides and LWR mixed oxide fuel and fuel from reprocessed uranium supply services to a number of other countries. This ongoing experience illustrates the option to avoid broad dissemination of the proliferation sensitive chemical reprocessing technologies by concentrating the production on a limited number of sites that could provide recycling services to multiple customers abroad.
The reactors operating (or potentially operating) under this option include LWR (and optionally HWR, very high temperature reactors (VHTR) and supercritical water cooled reactors (SCWR).
Option D. Complete recycle of spent fuel
With the use of a closed fuel cycle and breeding of fissile material all natural resources of fissile (235U) and fertile (238U) uranium and thorium (232Th) could eventually be utilized through the conversion of all fertile nuclear materials into fissile with their subsequent fission. This option realizes nearly full utilization of the energy potential in nuclear fuel. This enhancement option also reduces the long-lived radiotoxicity burden of HLW by up to an order of magnitude by keeping plutonium out of the waste.
If Option D is fully implemented, the use of previously mined uranium currently in used nuclear fuel and depleted uranium stocks solves the fuel resource utilization issue by providing fuel for very long periods of time (>1000 years) without any additional uranium mining. This enhancement also helps to achieve the Brundtland Commission objectives for utilization of non-renewable resources[2]. While the current generation will use some amount of current resources, they also will enable future generations to extract more energy from the remaining resources than was used by all previous generations combined.
This option requires the development and deployment of breeder or break-even (breeding ratio ~1) reactor technology, but reduces or eliminates the need for proliferation sensitive uranium enrichment.
This option may be based on either uranium or thorium as a source of fertile materials, including materials from LWR spent fuel. However, this option can only be achieved in the very long term as its deployment will — in many strategies — be constrained by the availability of plutonium or 233U from spent nuclear fuel and may require a large share of fast reactors (up to 60% or more of the total nuclear fleet). However, to become a reality in the future, relevant technologies need to be developed at the present.
Like Option C, Option D (complete recycle of spent fuel) could avoid broad dissemination of the proliferation sensitive wet or dry chemical reprocessing technologies by concentrating the production on a limited number of sites that could provide recycling services to multiple customers abroad not having the chemical reprocessing plants domestically, under the international nuclear trade framework.
The reactors that could operate under this option include, but are not limited to, any breeder or break-even and burner reactors that use excess fissile material from the breeder reactors. Therefore, they could include LWR, HWR, fast reactors, lead cooled fast reactors, gas cooled fast reactors, VHTR and molten salt reactors (MSR).
Option E. Minor actinide or minor actinides and fission products transmutation
A closed fuel cycle recycling all actinides and only disposing fission products would provide the maximum benefits for combined resource utilization and waste hazard minimization. This enhancement option builds on the technologies of the previous options, but also requires the development and deployment of minor actinide reprocessing/partitioning, minor actinide bearing fuels/targets, and remote fuel/target fabrication technologies.
A couple of decades ago, an option to transmute, along with minor actinides, also long-lived fission products was considered. As it was found that long term radiotoxicity of long lived fission products is much less than that of the minor actinides, further research along this trend faded.
The nuclear installations that could be used under Option E (minor actinide or minor actinides and fission products transmutation) include fast reactors, accelerator driven systems (ADS) and MSR. While a substantial progress with RD&D on such innovative technologies is noted in some Member States, the realistic deployment dates for such facilities are in the second half of the present century.
Option F. Final geological disposal of all wastes
Option F (final geological disposal of spent nuclear fuel/high level radioactive waste) applies to all Options A–E above. In this context, each generic fuel cycle option can be amended by adding Option F (e.g. AF, BF, CF and so forth) and only with such amendment could they be considered for sustainability.
The disposal material would however be different for different options. For Options A–C, it would be spent nuclear fuel (plus HLW for Option C), while for Options D and E it would only be HLW. For Option D, this would be a mixture of fission products and minor actinides; for Option E, a combination of fission products, potentially, without all or some long lived fission products and minor actinides.
While current NES include provision for future disposal of all wastes, and several final repository projects are under way, there are no operating disposal facilities for spent nuclear fuel or HLW. Option F addresses the political issue of waste management through the disposal of spent nuclear fuel and/or HLW. Retrievability of spent nuclear fuel and HLW may be required to not limit options for future generations, who may wish to use the spent nuclear fuel as a fuel resource or to implement improved HLW management options.
Direct spent nuclear fuel disposal may have additional long-term safeguards and security implications. For example, with respect to the disposal facilities in the ongoing repository projects, prior to the facility closure there will be a 100 year open operation period with the safeguards approach for this period already defined. However, according to provisions of comprehensive safeguards agreements, safeguards on nuclear material can only be terminated if it is determined to be unrecoverable, but that may not be the case after closure of the facilities. Therefore, there would be 100 years to figure out exactly how to apply safeguards for the very long term. Currently, there is no agreement on how to proceed in the long term, but concepts are being studied.
Under Options C–E, where large scale spent nuclear fuel reprocessing, plutonium storages and handling and plutonium fuel fabrication will be required, the most stringent safeguards and security measures on bulk and item material inventories that do not exist under Options A and B would need to be implemented. However, a practical example of safeguards implementation for industrial scale spent nuclear fuel reprocessing exists.
Sustainability Option A is fundamental to any sustainable NES. Options B–E can progressively improve resource sustainability of an NES while also substantially reducing the long-term waste burden. This may in turn facilitate the achievement of Option F (final geological disposal of all wastes). However, care needs to be taken that the advanced technologies and infrastructure deployed do not significantly increase overall costs. Competitive economics versus other energy options is, and would remain, an important driver for nuclear energy development, along with national and international considerations such as diversification of resources or environmental objectives such as greenhouse gas emission reduction. It could also be noted that moving from Option AF to Option EF may be a dynamic process involving multiple countries and partnerships.
Figure 2 presents a schematic for combining technical options in a synergistic manner to enhance the sustainability of the NES. Such technical synergies can be considered by countries with advanced technical capabilities and large enough national NES. In the case of other countries, a combination of technical options and collaboration with advanced countries would be the only feasible option.
It should be noted that even for the essentially ‘technical’ synergies that can be achieved within a single large national nuclear energy programme, cooperation with other countries would, in most cases, be helpful to enable, accelerate or optimize these ‘technical’ synergies.
One of the effective solutions to bring the benefits of enhanced sustainability options to a broader variety of users is through cooperation between countries in fuel cycle operations. This has been shown in previous INPRO studies[1] to reduce the technology development and infrastructure needs of newcomers and countries with smaller nuclear energy programmes, while assisting other cooperating countries in acquiring the benefits of enhanced sustainability options. The infrastructure basic principle in the INPRO methodology includes the development and provision of regional and international arrangements. Analysis of benefits of cooperation in the nuclear fuel cycle was one of the objectives of the SYNERGIES collaborative project. The project looked for options to amplify the benefits of innovation through synergistic cooperation in nuclear fuel cycles.
All of the INPRO sustainability subject areas can be enhanced through collaboration. Safety is improved through the exchange of not only advanced technology, but also information and knowledge on safety requirements and design certification, as well as human resources. Proliferation resistance and nuclear security can be improved by limiting the number of sites that employ enrichment and reprocessing technologies and associated fissile material stocks. Economics and infrastructure are improved through judicious investments in research and development and improved learning and economies of scale of larger fuel cycle facilities. Security of supply could be improved via political arrangements. Finally, the environment and waste management areas are improved by enabling more countries to achieve the benefits of enhanced NES sustainability options, e.g., through the use of fuel cycle services provided by countries with recycling capabilities or through multilateral solutions for waste repository.
It is important to note that nuclear trade and cooperation is substantively different from trade and cooperation in many other fields in that it is more stringently regulated, i.e., governed by the agreements between countries and between countries and the IAEA. These “agreements for peaceful nuclear co-operation” have more complex terms, restrictions and obligations than found under agreements governing trade of general commodities, goods and services. The current status of international trade and cooperation on nuclear power and nuclear fuel cycles is highlighted in the following paragraphs.
The current nuclear trade regime is governed predominantly under bilateral agreements for peaceful nuclear co-operation (hereafter referred to as “bilateral agreements”). These agreements are typically umbrellas that cover the terms of nuclear trade between two trading States in a broad sense including generic legal terms, restrictions and obligations that will apply to all captured trades and activities (e.g. so-called “deemed exports” such as intellectual property transfers) between the parties during the term of the agreement. Often, the terms of bilateral agreements are reciprocal between the parties so that both sides have equal legal terms and obligations, though on occasion there may be some asymmetry if agreed to between the sides. Beneath these bilateral agreements are so-called “subsequent arrangements” typically provided as attachments to the agreements, which define additional terms and obligations that apply to specific trades, captured items and activities associated with the bilateral agreement[15]. Beneath the bilateral agreements and subsequent arrangements there are contracts which are required to comply with the terms of these legal trade instruments.
Typically, a State establishes its nuclear trade by negotiating and concluding a series of bilateral agreements with its various supplier and customer States. Thus, the legal structure of trade evolves to meet the needs of a State’s growing nuclear industry through both import and export as the situation may require. This provides for secure supplies to a State, but also commonly conveys obligations, including those associated with safety, security and non-proliferation, to remain in compliance with the bilateral agreements. As nuclear materials, services, and equipment are accumulated or distributed through trade, bilateral agreement obligations are also accumulated and distributed. As a result, a sophisticated structure of international legal interdependence forms between States that are legally co-operating on nuclear energy. However, it is important to note that national policy, laws, regulations on import and disposal of foreign radioactive waste and SNF, provisions on transfer and reprocessing of the nuclear material in the bilateral agreements, the disparities between partners on the RD&D capacity, expertise, infrastructure, manpower, and financing could all act as impediments for effective implementation of international trade and cooperation on nuclear power and nuclear fuel cycle.
In rare cases, there are broader multilateral co-operation agreements. A most notable and sophisticated example is the “Treaty establishing the European Atomic Energy Community” (hereafter referred to as the “Euratom Treaty”), signed in 1957[16]. The Euratom Treaty created a common nuclear marketplace and now extends to include all members of the European Union.
If considered superficially, agreements governing international trade and cooperation on nuclear power and fuel cycle may seem to hamper competitive trade as found in less regulated markets. However, peaceful nuclear energy development and trade implies transfer of considerable and unique responsibilities and liabilities. The sophisticated nuclear trade regime helps to manage these specific and unique risks associated with nuclear energy development. Although rare, some examples of multilateral agreements (e.g. Euratom Treaty [V.13]), as well as the emerging multiplicity of suppliers and bilateral agreements among certain countries (nuclear power plants, fuel supplies and services) indicate that benefits of competitive trade can be achieved in the future for a variety of supplies in nuclear power and the nuclear fuel cycle, within the established governance models of international nuclear trade and cooperation. International cooperation is also viewed as crucial in developing the next generation of nuclear reactors.
The SYNERGIES case studies
Solutions to address the challenges for sustainability enhancement of regional and global nuclear energy systems were looked for and analysed in the case studies performed within the SYNERGIES project. The participating teams conducted in-depth country studies, each exploring longer term evolution of nuclear energy development in the respective country, considering synergies among the various technologies and options for regional and global cooperation. Altogether 27 case studies were carried out. These case studies analysed a number of scenarios built around the SYNERGIES storyline but combined different technical options and collaboration possibilities relevant to their specific conditions and national goals. Among these, 21 case studies explicitly addressed synergies in technology, 20 case studies addressed synergistic collaboration in nuclear fuel cycle back end with a link to synergies in technology, and 12 case studies touched upon possible cooperative solutions on regional or global level. Most of these case studies used IAEA’s model MESSAGE-NES[17] as the modelling tool for developing and analysing scenarios for nuclear energy systems.
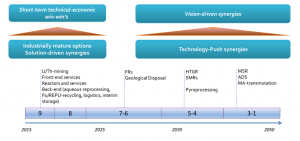
Figure 3 provides an illustration of the nature of the synergies explored in these case studies towards NESs with enhanced sustainability. Within the first timeframe shown in Fig. 3 synergies are mostly driven by ‘win-win’ situations defined by the present day technical-economic solutions. Within the third (last) timeframe, and to a certain degree also within the second (intermediate) timeframe, a more ’vision driven’ approach is explored towards enhancing sustainability of NESs to be boosted by technology push actions from innovative technology developers.
Besides evaluating the benefits of different synergistic options, the case studies also critically analysed the drivers that would persuade a country following a particular synergistic approach and identified the potential impediments for implementing such an approach. These investigations could help design action plans or roadmaps aimed at enhancing sustainability of nuclear energy systems.
Cooperative solutions on regional/global levels
Combination of national nuclear energy programmes and international cooperation
The case studies from a number of participating countries looked at possibilities for connecting domestic nuclear energy programmes with international cooperation aimed at solving long term problems in enhancing sustainability of nuclear power. They also attempted to find an optimal balance between their own capabilities and those provided by the external partners. Several of these countries have already demonstrated some common approaches to regional and interregional cooperation. The case studies from Armenia, Argentina, Indonesia, Romania and Ukraine are presented as examples.
The governments in these countries consider nuclear power as a stable component of the national energy mix taking into consideration security of supply, reliability, economic efficiency and low Green House Gas (GHG) emissions. Public opinion in these countries also supports further deployment of nuclear power. All countries from this group intend to further expand the use of nuclear power. They consider regional solutions for spent fuel repository as a promising development. These countries have on-going R&D programmes for nuclear power development and are already working on several steps of nuclear fuel cycles.
Argentina has local capacities of all the stages of the once-through nuclear fuel cycle and is developing small modular reactors (SMR) of local design. Argentina plans to become a regional supplier of nuclear power technology in Latin America and even a global supplier of the Argentinian CAREM SMR (Fig. 4).
The Argentinian team elaborated two scenarios for future development of nuclear energy in the country which involve the use of NPP supplied from abroad and locally designed NPPs and full utilization of the local fuel cycle capabilities. It was, however, found that locally available uranium reserves will be insufficient for future expansion of nuclear power and the import of uranium would have to be increased considerably. Continuation of local R&D combined with participation in international R&D projects, such as Generation-IV International Forum, was found to be the key for nuclear power development in Argentina in a sustainable manner.
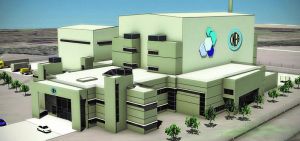
Romania has two CANDU 6 type nuclear power reactors in operation at Cernavoda NPP. Its current policy is a once-through nuclear fuel cycle based on indigenous facilities, without enrichment or reprocessing which are prohibited by the national laws. The spent nuclear fuel is stored at interim wet and dry storage facilities. A Near Surface Repository with multiple barriers has been built for final disposal of low and intermediate level waste (LILW) from the Cernavoda NPP. Research is being carried out on the geological environment for deep geological repository for spent nuclear fuel and high-level waste (HLW).
The Romanian team analysed three scenarios for future development of nuclear power - a) Basic case: four heavy water reactors (HWR), two existing and two new units; b) Pessimistic case: only two existing units; and c) Optimistic case: four HWR and another advanced reactor (a 1000 MW(e) PWR or an advanced HWR). Using IAEA’s MESSAGE model, the team calculated for each scenario the projected nuclear share in total electricity generation, annual nuclear fuel requirements, annual and cumulative uranium consumption, annual discharged spent fuel and spent fuel in interim wet and dry storage facilities, and the total investment requirements for nuclear power development up to 2050.
It was found that advanced HWR was the best economic option followed by advanced PWR. With the addition of two units each of the advanced HWR and PWR, the nuclear capacity would reach 3.8 GW(e), providing about 45% of the electricity demand in 2050. The local uranium resources would be sufficient for fuelling the HWR reactors the fuel for which will be produced at the local front-end fuel cycle facilities. For advanced PWR and advanced HWR, the fuel would have to be imported. The spent nuclear fuel from HWR will be kept in an interim dry storage, with cumulative amount reaching up to 8 kt HM (Fig. 5), while the spent nuclear fuel from the advanced PWR would be stored outside of the country in a regional storage facility. The Romanian case study incorporates international cooperation in the supply of reactors and fuel cycle services, including the spent nuclear fuel storage for advanced PWR.
The case study from the Ukraine explored the use of nuclear energy for co-generation of electricity and heat. Scenarios with introduction of SMRs and super critical water reactors (SCWR) were developed with the IAEA’s MESSAGE model. The scenarios assumed a once-through nuclear fuel cycle with spent nuclear fuel disposal. The results have shown that the SCWRs are more economic compared to advanced LWRs and would allow a decrease in spent nuclear fuel accumulation (Fig. 6). The development and deployment of a SCWR fleet would, however, require international cooperation in the nuclear fuel cycle field, particularly for fuel enrichment and fuel pellet sintering. As for the nuclear fuel cycle back end, it might be reasonable to address the capability of establishing a regional complex for long term spent nuclear fuel storage, so as to optimize the economic expenditures and minimize the deployment of dry spent nuclear fuel storage facilities at each NPP.
Another interesting case study exploring synergies in international cooperation was performed by experts from Indonesia. Presently, Indonesia does not have nuclear power plants but on a number of occasions has announced its intention to build NPPs based on foreign designs, while continuing with the domestic R&D programmes. The Indonesian study aimed at assessing the most viable option of fuel cycle strategies to support the sustainability of a nuclear energy programmes’ implementation in Indonesia, based on a potential of national, regional and international arrangements for the nuclear fuel cycle. Five nuclear fuel cycle options were evaluated (Fig. 7):
- (a) Once through fuel cycle involving 1000 MW(e) PWR with conventional uranium oxide (UO2) fuel and direct disposal of the spent nuclear fuel in a geological repository;
- (b) Plutonium mono-recycle with mixed oxide (MOX) fuel in PWR involving conventional reprocessing of LWR fuel, recycling of separated plutonium (Pu) in the form of U-Pu MOX fuel in PWRs, disposal of the HLW resulting from the reprocessing and direct disposal of the MOX spent nuclear fuel in a geological repository;
- (c) Direct use of PWR spent fuel in a CANDU reactor (an HWR) involving fabrication of the CANDU fuel from the spent PWR nuclear fuel without chemically separating the fissile material and fission products;
- (d) Synergistic fuel cycle LWR– fast reactors involving fast reactors to burn both 235U and transuraniums (TRU). The spent nuclear fuel of PWR will be reprocessed to obtain TRU-bearing fuel for fast reactors, while the remaining uranium partitioned from the spent fuel of PWR will be disposed as LILW. The TRU fuel after burning in a fast reactor will be repeatedly reprocessed through pyro-process, and the recovered TRU would be recycled in a fast reactor to close the nuclear fuel cycle; and
- (e) Once-through Th-U fuel cycle in PWR.
The case study has concluded that a once-through UOX fuel cycle with PWR would be the most viable option to support a nuclear power programme in Indonesia in a sustainable manner. If the available uranium resources become scarce (or if there are problems with spent nuclear fuel management), a limited recycle option with a single recycle of MOX in PWR could be considered. This would offer some uranium resource saving and a reduction in the waste production per unit of energy generated. However, this fuel cycle poses larger proliferation risk due to substantial working inventory of separated plutonium. Coupled with fast reactors (PWR-fast reactor strategy), this fuel cycle could become more attractive in the long-term.
- Fig. 7. Fuel cycle options considered in Indonesian case study.
Yet another example is the case study from Armenia. This case presented an approach to minimization of R&D and investments in the nuclear fuel cycle infrastructure deployment by means of cooperation with regional or transregional nuclear technology holders. Armenia uses a once through nuclear fuel cycle for its existing WWER-440 nuclear unit. The spent nuclear fuel, after being kept for 5 years in the reactor cooling pool, is transferred to dry storage. In search of a long-term solution for spent nuclear fuel management, the Armenian team evaluated three different options and calculated the impact of the spent nuclear fuel management cost on the cost of electricity generation. The three options considered were: (i) spent nuclear fuel storage at the NPP site; (ii) spent nuclear fuel storage at the NPP site and removal of the spent nuclear fuel from NPP site to geological disposal; (iii) Export of spent nuclear fuel from Armenia for reprocessing and final disposal in another country.
The study found that levelized fuel electricity cost of the final stage of the nuclear fuel cycle was only a small fraction (4%) of the total electricity generation cost . More importantly, the cost of different options for spent nuclear fuel management was found to have an insignificant effect on the total electricity generation cost (LUEC). Consequently, the study concluded that the option with the construction of the spent fuel dry storage was an acceptable solution to management of the spent nuclear fuel. However, given the need of spent nuclear fuel management after the project period of the storage in the spent fuel dry storage, export of spent nuclear fuel might be more attractive after its discharge from the reactor’s cooling pool. Appropriate cooperation arrangements would be needed for implementing such an approach.
Regional collaboration
Strong collaboration among countries on nuclear power is already functioning well in some regions, e.g., among the European Union (EU) members and among some Commonwealth of Independent States (CIS) countries. Such regional cooperative arrangements can further be expanded for enhancing sustainability of nuclear energy systems in each participating country as well as at regional and global levels.
The case study named ‘EU27 scenarios’ looked at the extended use of regional nuclear fuel cycle centres - the La Hague and MELOX facilities - for synergistic collaboration among the EU countries. The study examined the option of limited reprocessing of LWR spent fuel and fabrication of MOX fuel supply for a single recycle in LWRs. The results showed that a 10% to 15% reduction in natural uranium consumption together with minimization of waste could be achieved through such fuel cycle services. This would also reduce the spent nuclear fuel in interim storage (Fig. 8) and the requirements for deep geological disposal. Altogether, these factors may outweigh the associated slight increase of fuel costs. If the natural uranium prices increase in the future, this option would become economically more attractive.
The case study performed by experts from Armenia, Belarus, the Russian Federation and Ukraine analysed the benefits of continuation and extension of the regional collaboration already existing among these countries for the deployment and operation of WWER (Fig. 9).
In the scenario developed in this case study the LWR reactor fleet was supplemented by fast reactors in order to evaluate the prospects of the two-component nuclear energy system. The study has concluded that collaboration of technology holders and users in the back end of the nuclear fuel cycle is likely an inevitable solution on the path towards regionally/globally sustainable nuclear power. The following drivers for ‘win-win’ cooperation have been identified: exclusion of accumulation of the spent (also referred to as “used”) nuclear fuel and Pu therein; substantial reduction of U consumption (by a factor of 10 or more); use of the cheaper categories of U (e.g., depleted or reprocessed uranium), savings of financial and manpower resources for the user countries and expansion of nuclear energy business for the technology holders.
Another regional case study conducted by the Italian team explored a rather new type of regional cooperation. It examined the potential of co-operation between Italy and some European countries. Italy has a moratorium on nuclear energy and cannot build and operate a NPP on its territory; however, the Italian utility is involved in operation of several NPPs abroad. This study looked at the possibility of building a fleet of NPPs outside Italy in neighbouring countries, where the public stance regarding nuclear energy is not negative.
This possibility of regional cooperation emerges from two recent developments. The first one is the fast growth of high voltage lines interconnecting the European national electrical grids, triggered by huge increase in installation of intermittent renewable electricity sources (wind and PV), and the second one is the inevitable need for carbon free electricity generation to combat climate change. With this backdrop, the countries that, due to public opinion pressure, can’t build new NPPs on their territory might find it profitable to produce baseload nuclear electricity abroad.
The Italian case study estimated the benefits from operating a fleet of PWRs outside Italy, and subsequently at a proper time, introducing the innovative lead cooled fast reactors as well. The analysis indicated that the fuel costs could be cut down by 34–49% due to economies of scale in fuel enrichment and spent fuel reprocessing services. Besides the economic benefits, environmental benefits were also identified, such as complying with the European limitations on CO2 emissions. Additionally, there would be noticeable reduction of natural uranium consumption and waste disposal capacities.
Technical synergies among reactor technologies
Technical synergies aimed at plutonium utilization and prospects for international cooperation in this area
The case studies by expert teams from China, France, Japan, the Russian Federation and the Ukraine, as well as the study jointly implemented by IAEA experts and the Russian team, explored several variants of the technical synergies among thermal and fast reactors aimed at plutonium utilization and the related potential for international cooperation in the fuel cycle back end for enhancing sustainability of the regional and global NES. The studies essentially aimed at addressing the problem of spent nuclear fuel accumulation from PWRs and decreasing the natural uranium consumption based on possible closed nuclear fuel cycle scenarios.
The French case study explored different ways of utilizing the recovered plutonium in thermal and fast reactors. Presently, the NES in France is based on thermal reactors with uranium fuel and partial-loading of MOX-fuel with a single recycling of plutonium. In the future, plutonium can come from different sources but the content of plutonium in fresh LWR-MOX fuel must remain under 12 % for safety reasons. Introducing fast reactors can help maximize the utilisation of plutonium. This would, however, require a large scale deployment of fast reactors utilizing their own plutonium with a start-up on plutonium recovered from the thermal reactor spent fuel, not possible in the short and medium term.
The French case study has shown that plutonium multi-recycling would be possible with a symbiotic fleet composed of PWR-UOX, PWR-MOX and fast reactors optimized to reach equilibrium between plutonium consumption and production. This scenario minimized the number of fast reactors while maximizing the energy produced with LWRs-MOX using plutonium resources as fuel. The plutonium produced in UOX-PWRs is used to feed PWR-MOX as it is the case in France, and sodium cooled fast reactors are used to recycle plutonium from spent PWR-MOX fuel to improve its quality so it can finally be used together with spent UOX-fuel to produce fresh PWR-MOX fuel (Fig.10).
The Russian case study considered a slightly different approach with two steps in the LWR/ sodium cooled fast reactor system deployment (Fig.11). The first step involved a single-run use of accumulated plutonium from WWER in a small number of BNs (Sodium cooled fast reactors of Russian design). At this step BN serves as an ‘utilizer’ of WWER plutonium, realizing its energy potential and converting WWER fuel into a more compact form with improved isotopic composition of plutonium allowing longer storage without substantial americium-241 build-up.
The second step includes reprocessing the MOX spent nuclear fuel from BN reactors and using separated Pu for fabrication of the start-up fuel loads of the sodium cooled fast reactors operating in a fully closed nuclear fuel cycle. This arrangement would be suitable for a situation with high demand for nuclear energy. In case of stabilization of the demand for nuclear energy, a balance between production and consumption of plutonium could be achieved by multiple recycling of MOX-fuel in a system of WWER and sodium cooled fast reactors (Fig. 11, c).
The case study from China examined the potential of indigenously developed sodium cooled fast reactors under the four scenarios of plutonium multi-recycling. It was shown that to meet high national nuclear deployment targets intensive RD&D efforts would be required to develop and implement a metal fuelled sodium cooled fast reactor with a breeding ratio of above 1.4 and the associated advanced reprocessing technologies. The case study from Japan investigated possible role of the sodium cooled fast reactors and a closed nuclear fuel cycle in three national scenarios representing a reduction in the role of nuclear energy in the national energy mix, as required under the new energy policy after the Fukushima Daiichi nuclear power station accident in 2011. It was concluded that it would be advantageous to reprocess the spent nuclear fuel compared to its direct disposal and/or partial reprocessing.
The case study from the Ukraine evaluated LWR - sodium cooled fast reactor scenarios as a pathway to decrease spent nuclear fuel accumulation. The HWRs reactors operated on reprocessed uranium from LWR used fuel were also included into the model of national NES. In the medium term, a closed nuclear fuel cycle based on fast reactors was found to be economically unattractive for the Ukraine. The once-through nuclear fuel cycle based on HWR fed with regenerated uranium was found to be a better economical solution.
The joint study by the IAEA experts and the Russian participants looked into the major issues of transregional cooperation in the context of a future LWR/ sodium cooled fast reactor based NES. The heterogeneous world model and global scenarios developed in the GAINS collaborative project were used in the study[13]. It was assumed that commissioning of the LWR-MOX/fast reactor systems should be expected at first in a few countries mastering these technologies, delineated in GAINS as country group NG1 (nuclear strategy group 1). Then spent nuclear fuel from LWR of user countries (NG2 - experienced users, and NG3 - newcomers) could be sent to technology holder countries for reprocessing and fabrication of the MOX fuel to be used for fast reactors in technology holder countries and for LWRs in user countries (Fig. 12). Thus, international cooperation could make the advantages of the LWR/ sodium cooled fast reactor NES available and affordable to a wide range of nuclear power countries, including those who will not be able and willing to develop and deploy such systems domestically.
This case study identified several drivers for scientific and technical developments in the area of plutonium utilization. NES based on technical synergies among thermal and fast reactors makes it possible to stop further accumulation of the spent nuclear fuel from PWRs and the Pu therein or even to decrease the amount of plutonium to operational needs, with minimum storage capacities and minimal quantities of actinides accumulated during storage of the LWR spent nuclear fuel Fig. 13.
The demand for natural uranium in the LWR/sodium cooled fast reactor plutonium balanced NES can be reduced by a half in the case of stabilized nuclear energy demand or reduced down to 100 times in the case of high energy demand with a significant share of fast reactors in the system. Avoiding excessive plutonium accumulation would facilitate safeguards procedures in the fuel cycle back end. Absence of perceptible quantities of plutonium in radioactive waste is also a significant advantage from the standpoint of environmental impact.
Along with the drivers, several impediments were noted. Among these are a low pace of development and deployment of the LWR MOX and, especially, of the sodium cooled fast reactor MOX technologies, owing to present day availability of inexpensive large natural uranium reserves and high anticipated costs of fast reactor construction and spent nuclear fuel reprocessing and MOX fuel fabrication. The estimates of levelized fuel cycle unit costs are shown in Fig. 14.
Technical synergies aimed at waste management
The case studies from Belgium, Canada, China, France, Spain and the European Union investigated the potential for technical synergy with regard to waste management. Among these, two cases from Canada looked at options for (i) recycling of reprocessed uranium (REPU) from LWR in HWRs and (ii) re-burning of LWR americium in HWRs. The case on REPU recycling in HWRs indicated economic savings for HWR compared to natural uranium use under certain assumptions regarding natural uranium and reprocessing costs.
The economic value of REPU is the cost of fuel which would otherwise have to be obtained to produce the same total energy. Since fuel bundles made of REPU, or re-enriched REPU, displace normal fuel bundles, in each case the cost of natural uranium and the cost of fuel bundle assembly hardware are parameters which affect the value of the REPU. The economic values of REPU for direct recycling in an HWR and for re-enrichment (for use in an LWR) are shown in Fig. 15. At US $90/kg for cost of natural uranium, it was estimated that REPU from 33 MWd/kg used nuclear fuel is worth about US $230/kg if recycled in an HWR and US $100/kg if re-enriched for an LWR.
At a cost for reprocessing of LWR fuel between US $1000 and US $2000 per kg, the REPU route is not very attractive. However, if REPU is already available as a by-product of MOX production with no further costs, the low-burnup REPU supplied to an HWR in place of natural uranium would be an economic option. The main benefit of technical synergy between LWRs and HWRs is for management of waste. The americium from LWR generated spent nuclear fuel could be transmuted in HWRs. The averted disposal cost was estimated in the Canadian study, which is dependent on the cost of natural uranium, LWR fuel burn-up, cost of partitioning americium from LWR spent nuclear fuel and enrichment level of REPU. Figure 16 shows minimum required averted costs of HLW disposal for each storage duration and REPU enrichment.
The analysis has shown that implementation of this technical synergy would significantly reduce the decay heat of HLW, enabling a more efficient utilization of space in the long- term storage facilities and thus improving the sustainability of the nuclear energy system.
The French study dealt with radioactive waste transmutation options of long lived radioactive elements and analysed technical and economic scenarios considering the possibilities of optimization between the long-lived HLW transmutation processes, their interim storage and their disposal in a geological repository. The study has investigated six scenarios:
- Plutonium recycling in sodium cooled fast reactors (minor actinides are sent to the waste);
- Plutonium recycling and minor actinides (or Am alone) transmutation in sodium cooled fast reactors in homogeneous mode (minor actinides are mixed with reactor fuel);
- Plutonium recycling and minor actinides (or Am alone) transmutation in sodium cooled fast reactors in heterogeneous mode (putting minor actinides in radial blankets within a depleted UO2 matrix);
- Plutonium recycling in sodium cooled fast reactors and minor actinides transmutation in ADS.
The study has shown that the transmutation of minor actinides would significantly reduce their inventory in the geological repository, however, the minor actinide inventory in the reactors and reprocessing plants would increase (Fig. 17). The transmutation of Am and minor actinides would result in a reduction by a factor up to 7.3 (Am) to 9.8 (MA) of the footprint of the HLW disposal zone after an interim storage period of 120 years. Higher contents of minor actinides at the fuel manufacturing and reprocessing steps would require significant design modifications to deal with thermal and radiation protection problems. The complexity of the operations carried out during the operating phase (loading / unloading, interim waste storage, transport) would also increase.
Schemes involving the transmutation of all minor actinides are significantly impeded by the presence of curium, and their implementation constraints often exceed those of the scenarios in which only americium is transmuted. The economic studies conducted show that the cost increase related to the transmutation process could vary between 5 to 9% when sodium cooled fast reactors are used and 26 % in the case of ADS use.
The Spanish team studied economic implications of long term waste management options for European nuclear fleet under four scenarios spanning over 200 years.
- Scenario 1 (SCN-1) is the once through fuel cycle reference scenario;
- Scenario 2 (SCN-2) assumes that LWR reactors are replaced by LWR Gen-III reactors after year 2021, and by sodium cooled fast reactors after year 2040;
- Scenario 3 (SCN-3) is similar to Scenario 2 except that 56% of the sodium cooled fast reactor fleet utilizes minor actinide fuel for net transmutation, while 44% of the sodium cooled fast reactors burn only plutonium;
- Scenario 4 (SCN-4) assumes that minor actinides transmutation is performed exclusively with ADS units, while sodium cooled fast reactors are dedicated to plutonium burning and breeding.
The inventories of Pu and minor actinides accumulated over the study period in the four scenarios are shown in Fig. 18. It can be noted that introduction of sodium cooled fast reactors reduces significantly the amount of plutonium in the final repository down to 1% of the total. Minor actinides transmutation/incineration could be achieved with a strategy that includes sodium cooled fast reactors for both electricity generation and minor actinide transmutation, and with a strategy where sodium cooled fast reactors are used for electricity generation and ADS are essentially dedicated to minor actinide burning. The cost of electricity would however increase by 20-35%. Though the cost of HLW disposal would be reduced by a factor of 4 to 5, it would have a relatively small impact on levelized unit electricity cost.
The case study from China on minor actinide transmutation in sodium cooled fast reactors compared two fast reactor options to burn minor actinides produced by PWRs - one related to TRU fuelled sodium cooled fast reactor and the other one - a dedicated sodium cooled fast reactor burner. No ultimate winner was identified. The dedicated burner reactors are likely to have more safety related issues requiring further resolution, but they are more effective and could be deployed in relatively small numbers. The results suggest that cooperative development and ownership of such potentially more expensive burner reactors may be of benefit.
Drivers for and impediment to synergistic approaches
The drivers
Economic competitiveness of nuclear energy has been identified as the primary driver for cooperation among countries. The technology user countries and, especially newcomer countries, look for the solutions with minimum economic and financial costs. On the other hand, the technology developer countries, who are running large and costly research, design and demonstration (RD&D) programmes on innovative nuclear reactors and fuel cycles, look at strategic and business growth in anticipated national and world markets . The synergistic collaboration between technology developers and users could help exploit the economic benefits associated with the economy of scale of fuel cycle facilities and the economy of accelerated learning. Should such economies work, it could be a ‘win-win’ strategy for both.
The motivations of technology holder countries to pursue innovations in nuclear reactors and fuel cycles are inherently affected by the availability and cost of natural resources (e.g., uranium) and the situation with the progressive accumulation of spent
nuclear fuel from thermal reactors operated in a once through fuel cycle. At the moment, there are no sharp edges related to these, although progressive accumulation of spent fuel starts to press in places. With an increased role of these drivers for innovation, the motivation for both, technology holders and technology users, is to facilitate the deployment of these innovative technologies and services.
Other potential drivers for synergistic cooperation in sustainable NES, as identified in the SYNERGIES case studies, could be related to the solution of some public acceptance or social issues, such as the control of plutonium inventories in storage to reduce proliferation and security concerns, minimization of the amount of HLW to simplify siting acceptable geological disposal solutions with minimum environmental impacts and footprints, considerations of increased energy independence (non-reliance on natural uranium with its potentially volatile price), and preservation of natural resources (e.g. natural uranium for countries with large targeted nuclear programmes).
The SYNERGIES case studies indicate that some of the above-mentioned drivers may actually ‘work’ when the relevant disadvantages in economics are relatively small (a few per cent of the LUEC). However, the known current practice (the European Union’s LWR spent fuel reprocessing and MOX fuel supply for a single recycle in LWRs) indicates such collaborations are limited in scale and undertaken by more wealthy and experienced users. In the case of larger increases of global nuclear energy with the associated potential of resource insufficiencies, HLW accumulation and increased proliferation and security concerns, one could expect these public acceptance and social related drivers to work more effectively for synergistic collaboration targeted at nuclear energy sustainability.
The impediments
A number of impediments for exploiting the technical and collaborative synergies have been identified, including:
- National laws in some Member States prohibiting spent fuel transport across national borders;
- Non-available or insufficiently elaborated institutional procedures to govern nuclear fuel/ HLW transactions and price formation mechanisms for such transactions;
- National laws that permit the return of ultimate waste (e.g., fission products and minor actinides) only of the same isotopic content as in the originally exported fuel – this would hamper the operations of a large fuel cycle back end service provider or international fuel cycle centre for which it would be non-expedient to reprocess spent fuel individually for each customer;
- Regional directives narrowing the competition for reprocessing services, and, potentially many others .
Timely overcoming the above-mentioned impediments of institutions and infrastructure is a necessary step to enable synergistic collaboration among countries towards sustainable nuclear energy. Taking into account the time needed to change national laws and develop new institutional procedures may be a priority task for the near and medium term. The first step here would be to investigate the scope of legal and institutional issues in interested technology holder, technology user and newcomer countries more specifically and with higher degree of detail. Finally, political and economic instability are identified as impediments that could hamper cooperation among countries.
Near and medium-term actions towards enhanced nuclear energy sustainability
The basic concept of SYNERGIES with respect to sustainability is to have the whole achieve more than the parts. If one partner in a synergistic collaboration is achieving enhanced sustainability, then the other partner could achieve the same enhancement without the requisite investment in technologies and the related infrastructure. International cooperation could help to expand the benefits of innovative technologies to those users who have no plans to deploy them domestically.
Near and medium-term actions are needed to continue to ensure and improve the longer-term sustainability of global nuclear energy. Near and medium-term actions for technology development are focused on developing and demonstrating enabling technologies for the sustainability improvement options. The maturation of these technologies in the near and medium terms will help to enhance sustainability in the longer term, even if the technology holders only use them for domestic programmes. Use of these technologies in synergistic activities to assist other less developed programmes would further advance global sustainability. A key challenge for all advanced nuclear technologies is to improve economic performance.
Looking forward to managing growing SNF inventories in the near to medium term, geological repositories need to be opened for SNF disposal or reprocessing capacities need to be expanded and geological repositories opened for disposal of HLW. Either option will allow for reductions in SNF inventories while providing a waste solution missing from today’s NES.
The successful opening and operation of one or more repositories is likely to reduce public uncertainties about nuclear waste and improve the associated social attitudes concerning specific repository projects, enabling more rapid deployment later, including potentially the deployment of regional repositories accepting waste from multiple countries. Depending on waste acceptance criteria, the start up of repositories may also influence decisions on direct disposal versus reprocessing of SNF.
Insights on the synergistic approach and its implementation
Synergies among technological options related to nuclear power plant types and their fuel cycles (e.g. reprocessing or recycling of spent nuclear fuel as MOX, partitioning and transmutation) as well as collaboration policies of the technology suppliers and technology users and newcomer countries could be of benefit for speeding up transitioning to NESs of enhanced sustainability. However, collaborations would be viable only if based on a ‘win-win’ strategy for both suppliers and users (‘by sharing, we win together’). In this, the ‘not-invented-here syndrome’ from some technology holder or aspiring technology holder countries can hamper collaboration. Synergistic collaborations in fuel cycle back end offer higher rates of capacity growth and larger capacity centralized fuel cycle enterprises which would help to exploit the economy of learning and the economy of scale curves and support ‘win-win’ collaborative strategies through the resulting economic benefits for all.
Implementation of national or collaborative scenarios of nuclear energy evolution can face multiple impediments related to material imbalance at certain points in time or unavailability/uneven use of back end fuel cycle capacities. The potential presence of the impediments indicates that careful nuclear energy development scenario analysis is helpful in defining long term national nuclear energy strategies, as well as in assessing options of cooperation with other countries. To make the results of such analysis meaningful and practicable, careful collection and verification of the input data is needed.
Synergistic collaborations among countries in fuel cycle back end may be prevented or hampered by the impediments of institutional and infrastructure nature. Finding pathways to overcome such impediments is a necessary step to enable cooperating countries’ move towards sustainable nuclear energy. Taking into account the time needed to change national laws and to develop new institutional procedures such a resolution may be a priority task for the near and medium term. The first step here would be to investigate the scope of legal and institutional issues in interested technology holder, technology user and newcomer countries more specifically and with high degree of detail.
Participants of the INPRO Dialogue Forum on drivers and impediments for regional cooperation on the way to sustainable NESs, in Vienna in 2012, discussed synergistic approaches that would combine various NES options deployed within different countries into a global NES of enhanced sustainability. All participants embraced the idea that such synergistic development would and could be beneficial, though the drivers towards such development should primarily be induced by the current nuclear technology leaders (e.g. China, France, India, Japan, Republic of Korea, Russian Federation and United States of America).
Synergistic collaborations among technology holders and technology users in fuel cycle back ends are likely to start from services being provided by fuel cycle service vendors to the technology users under individual contracts governed by bilateral agreements between countries. In this, certain technology users might choose to have bilateral agreements with countries hosting certain vendors, and the fact that quite a number of vendors are likely to emerge in the near to medium term could secure a certain competition preventing the monopoly. However, at the Forum the representatives of newcomer countries indicated that an option of outsourcing of the back-end fuel cycle services was viewed as preferable only in the short term. In the medium and long term, they see an international solution for fuel cycle back end as preferable, to exclude any monopolistic or cartel arrangement approaches.
Conclusion
This brochure presents only the major findings and conclusions of the SYNERGIES collaborative project. The full report entitled Enhancing Benefits of Nuclear Energy Technology Innovation through Cooperation among Countries was published as IAEA Nuclear Energy Series No. NF-T-4.9[1] in 2018. The publication has several appendices with information useful for further studies of options to enhance nuclear energy sustainability.
Appendix I includes key indicators for collaborative NES scenario evaluation on sustainability used in the case studies. Appendix II presents economic assessment data, methods and tools. Appendix III provides a short description of the GAINS approach. Appendix IV summarizes major findings of the fourth INPRO Dialogue Forum, on drivers and impediments for regional cooperation on the way to sustainable nuclear energy systems. Appendix V introduces concept of options for enhanced nuclear energy sustainability.
Full reports of the case studies performed within the SYNERGIES project are included on the CD-ROM accompanying the publication[1].
References
- ↑ 1.0 1.1 1.2 1.3 1.4 1.5 INTERNATIONAL ATOMIC ENERGY AGENCY, Enhancing Benefits of Nuclear Energy Technology Innovation through Cooperation among Countries, Final Report of the INPRO Collaborative Project on Synergistic nuclear energy regional group interactions evaluated for sustainability (SYNERGIES), IAEA Nuclear Energy Series NF-T-4.9, (2018)[1]
- ↑ 2.0 2.1 BRUNDTLAND COMMISSION, World Commission on Environment and Development, “Our Common Future”, Oxford University Press, Oxford (1987)
- ↑ 3.0 3.1 UNITED NATIONS, Conference on Environment and Development, Vol. I, Resolutions Adopted by the Conference (United Nations Publication, Sales No. E.93.I.8), Rio de Janeiro (1992)
- ↑ UNITED NATIONS, World Energy Assessment, “Energy and the Challenge of Sustainability”, (United Nations Publication, Sales No. OO.III.B.5), New York (2000).
- ↑ UNITED NATIONS, The Future We Want, Outcome document of the United Nations Conference on Sustainable Development, Rio de Janeiro, Brazil, 20–22 June 2012
- ↑ UNITED NATIONS, Transforming our World: the 2030 Agenda for Sustainable Development, 2015: [2]
- ↑ 7.0 7.1 INTERNATIONAL ATOMIC ENERGY AGENCY, Guidance for the Application of an Assessment Methodology for Innovative Nuclear Energy Systems. IAEA-TECDOC-1575, Vienna (2008)
- ↑ 8.0 8.1 INTERNATIONAL ATOMIC ENERGY AGENCY, INPRO Methodology for Sustainability Assessment of Nuclear Energy Systems: Infrastructure: A report of the International Project on Innovative Nuclear Reactors and Fuel Cycles (INPRO) IAEA Nuclear Energy Series NG-T-3.12, Vienna (2014).
- ↑ 9.0 9.1 INTERNATIONAL ATOMIC ENERGY AGENCY, INPRO Methodology for Sustainability Assessment of Nuclear Energy Systems: Economics: A report of the International Project on Innovative Nuclear Reactors and Fuel Cycles (INPRO) IAEA Nuclear Energy Series NG-T-4.4, Vienna (2014).
- ↑ 10.0 10.1 INTERNATIONAL ATOMIC ENERGY AGENCY, INPRO Methodology for Sustainability Assessment of Nuclear Energy Systems: Environmental Impact from Depletion of Resources: A report of the International Project on Innovative Nuclear Reactors and Fuel Cycles (INPRO) IAEA Nuclear Energy Series NG-T-3.13, Vienna (2015).
- ↑ 11.0 11.1 INTERNATIONAL ATOMIC ENERGY AGENCY, INPRO Methodology for Sustainability Assessment of Nuclear Energy Systems: Environmental Impact of Stressors: A report of the International Project on Innovative Nuclear Reactors and Fuel Cycles (INPRO) IAEA Nuclear Energy Series NG-T-3.15, Vienna (2016).
- ↑ Analytical Framework for Analysis and Assessment of Transition Scenarios to Sustainable Nuclear Energy Systems, IAEA/INPRO Brochure, Vienna (2014)
- ↑ 13.0 13.1 13.2 13.3 INTERNATIONAL ATOMIC ENERGY AGENCY, Framework for Assessing Dynamic Nuclear Energy Systems for Sustainability, Final Report of the INPRO Collaborative Project on Global Architectures of Innovative Nuclear Energy Systems with Thermal and Fast Reactors and a Closed Nuclear Fuel Cycle (GAINS), IAEA Nuclear Energy Series NP-T-1.14, (2013):
- ↑ UNITED NATIONS, Our Common Future (Report to the General Assembly), World Commission on Environment and Development, UN, New York (1987).
- ↑ THE U.S. GOVERNMENT FEDERAL REGISTER, Subsequent Arrangement between the United States and India, Vol. 75, No. 95, May 18, 2010, Notices, p. 27768
- ↑ EUR-LEX ACCESS TO EUROPEAN UNION LAW, Consolidated version of the treaty establishing the European Atomic Energy Community; 2012/C 327/01
- ↑ INTERNATIONAL ATOMIC ENERGY AGENCY, Modelling Nuclear Energy Systems with MESSAGE: A Users’ Guide, IAEA Nuclear Energy Series No. NG-T-5.2, IAEA, Vienna (2016):
- ↑ U.S. DEPARTMENT OF ENERGY, Technology Readiness Assessment Guide, DOE G 413.3–4 10–12–09, Washington D.C. (2009) [www.directives.doe.gov]
- ↑ Advances in Small Modular Reactor Technology Development, A Supplement to IAEA Advanced Reactor Information System (ARIS), IAEA Brochure 2018