INPRO collaborative project: GAINS (Sustainability Assessment)
This page is the "Appendix III" to Environmental Impact from Depletion of Resources
During 2008–2011, INPRO conducted the collaborative project GAINS[1], with participation by Belgium,
Canada, China, Czech Republic, France, India, Italy, Japan, Republic of Korea, Russian Federation, Slovakia,
Ukraine, USA and the European Commission, with Argentina as an observer.
This appendix summarizes the findings of GAINS, focusing on the issue of demand for uranium resources by
possible NESs until the end of the twenty-first century.
Contents
Introduction
The overall objective of the GAINS project was to develop a standard framework — including a
methodological platform, assumptions and boundary conditions — for assessing future NESs, taking into account
sustainable development, and to validate the results through sample analyses.
It is to be noted that this project did not have a specific goal to evaluate the potential global supply of uranium
until the end of the century and beyond, but focused on the introduction of advanced nuclear reactor technologies
and associated fuel cycle strategies in a heterogeneous real world model, with the cooperation of different countries
in the nuclear fuel cycle to achieve a number of sustainability goals, including a reduction of the global demand for
uranium.
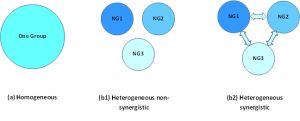
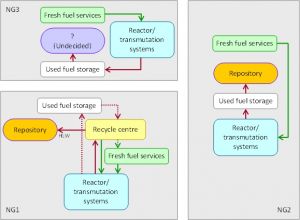
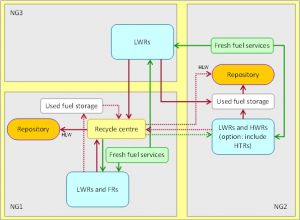
The project used two main models to investigate the possible development of nuclear power during the twenty-first century. One model employed the assumption of a complete homogeneous world, i.e. a world that involves uniform nuclear technology application in each and every country. A second, more realistic, approach was to define a heterogeneous world with three different groups (NG1–NG3) of non-personified countries with different policies regarding the back end of the nuclear fuel cycle. The three groups NG1–NG3 are defined as:
- NG1: General strategy is to recycle used fuel. This group plans to build, operate and manage FRs and use fuel recycling facilities and geological disposal facilities for highly radioactive waste (from reprocessing).
- NG2: General strategy is to either directly dispose of used fuel, or reprocess used fuel abroad. This group plans to build, operate and manage thermal reactors and geological disposal facilities for highly radioactive waste (in the form of used fuel and/or reprocessing waste) and/or work synergistically with another group to have its fuel recycled.
- NG3: General strategy is to use fresh fuel, and send used fuel abroad for either recycling or disposal, or the back end strategy is undecided. This group has no plans to build, operate and manage used fuel recycling facilities or permanent geological disposal facilities for highly radioactive waste. It may obtain fabricated fuel from abroad, and may arrange for export of used fuel.
In addition, in the three group approach, non-synergistic and synergistic behaviours of the world are assumed.
The selected models of the world are shown in Fig. 1.
A synergistic world requires communication and material flow between groups of countries, whereas a
non-synergistic world would not exchange nuclear materials other than fresh fuel. Figures 2 and 3 illustrate
non-synergistic and synergistic models of the world, respectively.
GAINS performed parametric evaluations of a payback time for investments in technology development for
FRs and a closed nuclear fuel cycle versus planned national deployment of such technologies. It was concluded
that, at least within the twenty-first century, the world is likely to follow the heterogeneous model shown in Fig. 3.
Several analytical tools were used in the GAINS study, but most analytical results were produced using the
computer codes MESSAGE, NFCSS and DESAE, available from the IAEA (brief descriptions of these tools can be
found in the overview volume of the updated INPRO manual). In many cases, participants in the study also used
their national computer codes (Commelini–Sicard (COSI) by France, Dynamic Analysis of Nuclear Energy System
Strategies (DANESS) and Verifiable Fuel Cycle Simulation Model (VISION) by the USA, and Tool for Energy
Planning Studies (TEPS) by India) to support the project. The GAINS report[1] includes a brief description and the
results of cross-verification of all these codes.
Global demand for power supply by nuclear energy
The GAINS project, inter alia, evaluated a series of available studies with nuclear power scenarios and documented a brief summary thereof in the final report[1]. The studies included: International Nuclear Fuel Cycle Evaluation[2], Joint Study: Assessment of Nuclear Energy Systems Based on a Closed Nuclear Fuel Cycle with Fast Reactors[3], and Nuclear Energy Development in the Twenty-first Century[4] by the IAEA; Nuclear Fuel Cycle Transition Scenario Studies[5], Strategic and Policy Issues Raised by Transition from Thermal to Fast Reactors[6], Advanced Nuclear Fuel Cycles and Radioactive Waste Management[7], and Trends in the Nuclear Fuel Cycle[8], by the OECD/NEA; Red Impact Synthesis Report by SCK·CEN[9]; and Partitioning and Transmutation European Roadmap for Sustainable Nuclear Energy by the European Commission[10].
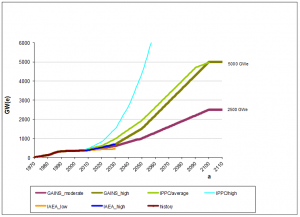
Based on comprehensive analysis and intensive discussions of the available projections on nuclear demand in the twenty-first century, GAINS selected two nuclear energy demand scenarios (as illustrated in Fig. 4):
- A high nuclear energy demand scenario, a variant of the medium expectation of the IPCC Special Report on Emission Scenarios. In this scenario, global annual nuclear energy generation reaches approximately 700 GW(e) by 2030, 1500 GW(e) by the middle of the century and 5000 GW(e) by 2100.
- A moderate nuclear energy demand scenario, assuming approximately 600 GW(e) by 2030, 1000 GW(e) by mid-century and 2500 GW(e) by the end of the century.
Types of reactors considered in GAINS
GAINS has developed an internationally verified database for material flow analysis of NESs with reactors and nuclear fuel cycles of different types. The reactor types used in basic calculations of GAINS correspond to proven thermal and FR technologies, leaving little doubt as to the feasibility of the corresponding nuclear power plants. The following types of reactors are included in the GAINS database:
- Light water cooled and moderated reactors (LWRs) with low, medium and high burnups (45 × 103–60 × 103 MWˑd/t) and different thermal efficiencies;
- Heavy water cooled and moderated reactors (heavy water reactors (HWRs)) with different types of fuel (UO2, ThO2, 233U and PuO2);
- FRs with different conversion factors (1.00–1.16) and different burnups (31–100 MWˑd/t).
In all cases, it was assumed that FRs were started from the U–Pu fuel load obtained from reprocessing of the SNF of LWRs.
Scenarios considered in GAINS
As mentioned previously, GAINS looked at homogeneous and heterogeneous scenarios, and for the latter, at
non-synergistic and synergistic behaviours.
For a homogeneous world, the following NES scenarios were considered: BAU+ (business as usual
with present day and advanced LWRs, with ‘+’ standing for the latter) and BAU+–FR (business as usual with
introduction of FRs). First, the results for the selected homogeneous scenarios will be presented, followed by the
heterogeneous non-synergistic and synergistic scenarios.
BAU+ scenario in a homogeneous world
The homogeneous scenario without introduction of FRs is presented here. The global NES in the
BAU+ scenario includes standard LWRs (as installed at 2008) and a conventional HWR, and after 2015, gradually
adds advanced types of LWRs called advanced light water reactors (ALWRs), with higher burnups and thermal
efficiencies, which completely replace the standard LWRs after 2055. The global power generation is shown in
Fig. 5[1] and the uranium consumption is shown in Fig. 6[1].
- FIG. 5. Power generation in the BAU+ scenario in a homogeneous world (high case on the left, moderate case on the right). ALWR — advanced light water reactor; HWR — heavy water reactor; LWR — light water reactor.
- FIG. 6. Total global uranium usage for the BAU+ scenario in a homogeneous world (high case on the left, moderate case on the right). ktHM — kilotonnes of heavy metal.
The total global uranium consumption reaches 36.1 × 106 t and 20.9 × 106 t by 2100 for the high and moderate BAU+ cases, respectively.
BAU+–FR scenario in a homogeneous world
The BAU+–FR scenario includes, in addition to FRs, standard LWRs (as installed at 2008) and conventional
HWRs, and after 2015, ALWRs with higher burnup, which completely replace the standard LWRs by 2055.
In this scenario, FRs (with a conversion rate of 1.0, a so called break even core design) — in addition to
standard LWRs and HWRs — are assumed to be initially introduced in 2021 at a low rate of 1 GW·a of power
generation per year for the first 10 years; after 2031, the installation rate is increased to 9.5 GW·a and 19.5 GW·a
for the moderate and high cases, respectively; after 2051, the installation rate is only limited by the amount of
plutonium available for the FRs and the overall growth rate.
Figure 7[1] shows the total power generation for the different types of reactors, and Fig. 8[1] shows the
global cumulative uranium consumption together with the limits from the Red Book 2009 (known and ultimate
resources)[11].
In this homogeneous scenario BAU+–FR, the total uranium consumption reaches, by 2100, values of
~25 × 106 tU and ~15 × 106 tU for the high and moderate cases, respectively.
- FIG. 7. Power generation for different reactor types in the BAU+–FR scenario in a homogeneous world (high case on the left, moderate case on the right). ALWR — advanced light water reactor; FR — fast reactor; HWR — heavy water reactor; LWR — light water reactor.
- FIG. 8. Total global uranium usage for the BAU+–FR scenario in a homogeneous world (high case on the left, moderate case on the right). ktHM — kilotonnes of heavy metal.
BAU+–FR scenario in a heterogeneous world
The heterogeneous world model extends the BAU+–FR homogeneous scenario by dividing the world into
three non-geographic groups, NG1–NG3, where the countries within a group all adopt the same fuel cycle strategy
(see Section III.1 above). The first nuclear power group (NG1) adopts recycling and a transition to FRs, as assumed
in the (global) homogeneous BAU+–FR scenario. NG2 continues with the BAU+ strategy of a once through
fuel cycle based on standard LWRs and HWRs, plus ALWRs, without recycling. NG3 starts to introduce LWRs
and HWRs beginning in 2008 and replacing them by ALWRs after 2015. The 6% share of global HWRs are all
modelled as part of NG2.
The heterogeneous cases use the same overall nuclear power demand curves for high growth and moderate
growth as the homogeneous cases. The contributions of each group NG1–NG3 to the global nuclear power
generation during the twenty-first century are based on extrapolation of data available in 2009 and on expert
evaluations carried out within GAINS: by 2100 — NG1:NG2:NG3 = 0.4:0.4:0.2.
As stated previously, in the heterogeneous model, a non-synergistic and a synergistic world were considered
in GAINS. The results of the non-synergistic scenarios in a heterogeneous world will be discussed first.
Heterogeneous BAU+–FR scenario in a non-synergistic world
For the non-synergistic world model, no movement of used nuclear fuel occurs between groups NG1 and
NG2 in this scenario. This limits the amount of LWR and ALWR spent fuel available in NG1 for reprocessing and
starting of FRs. An example of such a heterogeneous non-synergistic global NES was presented in Fig. 2 above.
The total power generation during the century globally and for three non-geographical groups are shown in
Fig. 9.[1]
Figure 9 illustrates the assumptions made for this scenario. The groups NG3 and NG2 install standard LWRs,
ALWRs and HWRs, and only group NG1 also installs FRs with a conversion rate of 1.0 (a break even FR design).
The combined power generation of the three groups is the same as that defined for the homogeneous case.
Figure 10 shows the cumulative uranium consumption of this non-synergistic heterogeneous scenario
BAU+–FR.
- FIG. 9. Power generation for different types of reactors and groups in the BAU+–FR scenario in a non-synergistic heterogeneous world. ALWR — advanced light water reactor; FR — fast reactor; HWR — heavy water reactor; LWR — light water reactor.
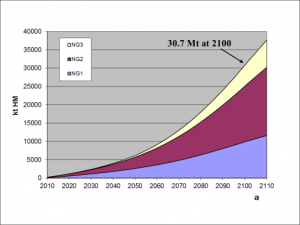
The value of global uranium consumption by 2100 for the heterogeneous non-synergistic scenario BAU+–FR (Fig. 10) is ~31 × 106 tU for the high case (and ~17 × 106 tU for the moderate case, not shown in Fig. 10). These values are between the values for the homogeneous BAU+ scenario (Fig. 6, ~36.1 × 106 tU for the high case and ~21 × 106 tU for the moderate case) and the values for the homogeneous BAU+–FR scenario (Fig. 8, ~24 × 106 tU for the high case and ~14 × 106 tU for the moderate case). This means that the full potential of FRs to reduce the need for uranium resources cannot be achieved in this scenario BAU+–FR. As stated above, this is caused by the non-availability of sufficient spent fuel to be reprocessed (to produce MOX fuel) in the group NG1 in this non-synergistic heterogeneous model.
Summary of selected scenarios considered in GAINS
A summary of results regarding the global uranium consumption for selected scenarios is presented in Table 1. In scenario 1, called BAU+, only thermal reactors (standard LWRs and HWRs) are assumed to be built during the twenty-first century plus ALWRs with increased burnups and higher efficiencies. Scenarios 2–4 include the introduction of FRs with different conversion rates and burnups into a global NES.
Scenario | Homogeneous model | Heterogeneous model
Non-Synergistic |
Heterogeneous model
Synergistic | ||
---|---|---|---|---|---|
Natural uranium demand by 2100 (× 106 tU) | |||||
1 | BAU+ | High moderate | 36.1
20.9 |
36.1
20.9 |
36.1
20.9 |
2 | BAU+-FR (CNR=1) | 24.1
13.4 |
30.8
17.2 |
26.3
14.9 | |
3 | BAU+–FR (CNR=1.2) | 21.2
11.3 |
29.4
16.2 |
26.3
14.9 | |
4 | BAU+–FR
(CNR=1.2, high burnup) |
22.1
11.8 |
29.9
16.5 |
26.3
14.9 |
Note: Case 1 corresponds to the scenario BAU+ presented in Section 4.1; case 2 corresponds to the scenario BAU+–FR presented
in Section 4.3; case 3 is similar to case 2, but for a conversion rate (CNR) of 1.2 in the fast reactors installed; case 4 is similar
to case 2, but for a CNR of 1.2 and a high burnup in the fast reactors installed.
In all cases, the homogeneous model shows the lowest global consumption of uranium by 2100 in comparison
with the heterogeneous models; therefore, the homogeneous model is obviously too optimistic. The non-synergistic
heterogeneous model demonstrates that the full potential of FRs to save uranium resources cannot be achieved
because no exchange of nuclear material between countries takes place in this model. The synergistic heterogeneous
model illustrates the significant potential of FRs to save uranium resources in a world that is freely exchanging
nuclear material, i.e. fresh and used fuel. As has already been mentioned, one of the main conclusions of the
GAINS project was that within the twenty-first century, the world is likely to follow the heterogeneous model, with
the degree of cooperation between countries (i.e. the degree of synergism) still being an open question[1].
Although GAINS has not introduced any limits on natural uranium resources (assuming that their extended
use would result in an overall uranium cost increase rather than physical depletion), the results of Table 7 could still
be analysed, just as an example, against the data on the natural uranium resources from the Red Book.
Based on the Red Book 2009, the identified resources of uranium could be defined as ~7 × 106 tU, and
the sum of all conventional resources as ~18 × 106 tU[11]. Then, if it is assumed that natural uranium from
conventional resources would have reasonably low costs and natural uranium from non-conventional resources
would have much higher costs, it may make sense to select 18 × 106 tU as a sort of a boundary against which the
Table 1 data could be analysed.
In all moderate cases discussed in GAINS, a global NES with predicted nuclear generation of 2500 GW(e)
by 2100 would most likely not run over the boundary of 18 × 106 tU during this century, especially if FRs are
introduced in a number of countries. However, this is not true for the high cases with a predicted nuclear generation
of 5000 GW(e) by 2100. To keep natural uranium consumption within the 18 × 106 tU boundary in cases of such
a high growth rate of global nuclear power would definitely require the timely introduction of FRs in a number
of countries throughout the world. On a more positive note, recent developments indicate that by around 2030,
commercial deployment of FRs is likely to start in a number of technology holder countries around the world.
It should be noted that very similar conclusions were obtained in an earlier IAEA study, Nuclear Energy
Development in the 21st Century: Global Scenarios and Regional Trends[12].
Conclusion
The GAINS project has developed a framework for analysing global NES architecture and has shown
— through sample analyses — that sustainability is more easily achieved on a global scale with collaboration
(synergistic approach) among countries with different policies regarding the back end of the fuel cycle.
In comparison to a non-synergistic approach, the synergistic approach with a worldwide free flow of nuclear
material can, inter alia, result in more effective utilization of fissile/fertile material resources in the global system
by making SNF available for recycling and reuse in nuclear reactors that otherwise might be disposed of as waste.
A synergistic approach could facilitate a partial solution to the problem of accumulating SNF inventories
and associated waste disposal in each individual country with a nuclear power programme. Countries with smaller
programmes could avoid developing their own nuclear waste management infrastructure by returning SNF to the
countries that supplied the fuel and who would recycle the returned fuel for further use in national FR programmes.
Given the current political situation, a high level waste facility would still be required; however, this would be for
disposal of fission products (only).
Regarding global uranium resources and consumption, it can be concluded that total conventional (ultimate)
resources of uranium (~17 × 106 tU as recorded in the Red Book 2009[11]) are almost sufficient to enable the
operation of a global NES based on advanced thermal reactors without running out of a fuel supply until the
end of the twenty-first century, if the total installed capacity of nuclear power at the end of the century does not
exceed 2500 GW(e) (called the moderate case in GAINS), which corresponds to about a sixfold increase by 2100
over the installed nuclear capacity in 2013. If the growth rate of nuclear power increases above such a value, a
timely introduction of FRs in specific countries that master this technology is capable of mitigating any shortage of
reasonably priced natural uranium.
It should be noted that non-conventional resources of uranium (see Appendix I) discussed in the Red
Book 2011 (~22 × 106 tU)<ref name=r13</ref>, e.g. in phosphates and black shale, which would be globally available at higher
prices of uranium mining would increase the available uranium resources considerably. If uranium from sea water
is taken into account (more than 4 billion tonnes), the global resource of uranium becomes practically unlimited.
It is also a known fact that uranium prices do not significantly influence the cost of electricity production by
nuclear power plants, as the uranium price is below 5% of the total electricity generation cost of a nuclear power
plant. The capital costs are the dominant factor at up to ~60%, operation and maintenance costs are ~25%, and the
remaining costs of the nuclear fuel of 15% include the costs of uranium, conversion, enrichment and fuel element
production. Thus, an increase of uranium prices might not be an obstacle for enlarging a global NES, at least with
nuclear power plants based on state of the art LWR technologies.
See also
[ + ] Assessment Methodology | |||||
---|---|---|---|---|---|
|
References
- ↑ 1.00 1.01 1.02 1.03 1.04 1.05 1.06 1.07 1.08 1.09 1.10 1.11 1.12 1.13 INTERNATIONAL ATOMIC ENERGY AGENCY, Framework for Assessing Dynamic Nuclear Energy Systems for Sustainability: Final Report of the INPRO Collaborative Project GAINS, IAEA Nuclear Energy Series No. NP-T-1.14, IAEA, Vienna (2013).
- ↑ SKJÖLDEBRAND, R., The International Nuclear Fuel Cycle Evaluation — INFCE, Int. At. Energy Agency Bull. 22 2 (1980).
- ↑ INTERNATIONAL ATOMIC ENERGY AGENCY, Assessment of Nuclear Energy Systems Based on a Closed Nuclear Fuel Cycle with Fast Reactors, IAEA-TECDOC-1639/Rev.1, IAEA, Vienna (2012)
- ↑ INTERNATIONAL ATOMIC ENERGY AGENCY, Nuclear Energy Development in the 21st Century, IAEA Nuclear Energy Series NP-T-1.8, IAEA, Vienna (2010).
- ↑ ORGANISATION FOR ECONOMIC CO-OPERATION AND DEVELOPMENT NUCLEAR ENERGY AGENCY, Nuclear Fuel Cycle Transition Scenario Studies, Status Report, OECD/NEA Rep. 6194, OECD, Paris (2009).
- ↑ ORGANISATION FOR ECONOMIC CO-OPERATION AND DEVELOPMENT NUCLEAR ENERGY AGENCY, Strategic and Policy Issues Raised by Transition from Thermal to Fast Reactors, OECD/NEA Rep. 6352, OECD, Paris (2009).
- ↑ ORGANISATION FOR ECONOMIC CO-OPERATION AND DEVELOPMENT NUCLEAR ENERGY AGENCY, Advanced Nuclear Fuel Cycles and Radioactive Waste Management, OECD/NEA Rep. 5990, OECD, Paris (2006).
- ↑ ORGANISATION FOR ECONOMIC CO-OPERATION AND DEVELOPMENT NUCLEAR ENERGY AGENCY, Trends in the Nuclear Fuel Cycle, OECD, Paris (2001).
- ↑ FORSCHUNGSZENTRUM JÜLICH, RED-IMPACT: Impact of Partitioning, Transmutation and Waste Reduction Technologies on the Final Nuclear Waste Disposal, Synthesis Report, FP6 Contract No. FI6W-CT-2004-002408, Forschungszentrum Jülich, (2007).
- ↑ EUROPEAN COMMISSION, Coordination Action of the European Commission Sixth Framework Programme, PATEROS: Partitioning and Transmutation European Roadmap for Sustainable Nuclear Energy, FP6 Contract No. FI6W-036418 SCK·CEN, Mol (2008).
- ↑ 11.0 11.1 11.2 ORGANISATION FOR ECONOMIC CO-OPERATION AND DEVELOPMENT NUCLEAR ENERGY AGENCY, INTERNATIONAL ATOMIC ENERGY AGENCY, Uranium 2009: Resources, Production and Demand, (‘Red Book’), OECD/NEA, Paris (2010), [1]
- ↑ INTERNATIONAL ATOMIC ENERGY AGENCY, Nuclear Energy Development in the 21st Century: Global Scenarios and Regional Trends, IAEA Nuclear Energy Series No. NP-T-1.8, IAEA, Vienna (2010).