Basic concepts for optimizing management options for reduction of environmental impact (Sustainability Assessment)
This page is the "Appendix III" to Environmental Impact of Stressors
As low as reasonably practicable
Concept
The concept of ALARP (economic and social factors taken into account) is used to define an AL for several INs in different areas of the INPRO methodology, especially for the environment and waste management.
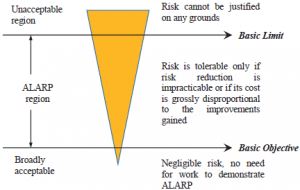
The concept is illustrated in Fig. 1. As shown, the risk (symbolized by the triangle) is divided into three
regions: a broadly acceptable region, a tolerable region where a process for ALARP has to be used and an
unacceptable region.
As a first step of the ALARP concept to be applied within the INPRO methodology, the boundary values of
these three regions have to be determined: the boundary between the tolerable and the unacceptable regions, usually
called a basic limit (BL); and the boundary between the tolerable and broadly acceptable regions, sometimes called
a basic objective (BO). The next step is to confirm that the value of the indicator of an NES is within the ALARP
region that is below the BL. The last step is to confirm that in an optimization analysis, all measures to reduce
the specific risk have been taken into account by the designer up to a level where the costs for these measures
would have become ‘grossly disproportional’ to the benefit gained. It is important to note that, in the case when
the indicator of an NES has a value in the broadly acceptable region below the boundary BO, no further effort is
necessary to fulfil the ALARP concept.
BL and BO values may be specified for specific indicators in national regulations or as an outcome of an
EIA, or it may be necessary to infer such values from other evidence such as: licence conditions; actions planned,
under way or completed to remediate an existing situation or improve a given practice; presentations at national
or international conferences; publications in peer reviewed journals, the IAEA Safety Standards Series and other
IAEA publications; and the work of other organizations such as the ICRP, the NEA and the European Commission.
When a BL or a BO is deduced from such evidence, the rationale for doing so needs to be clearly stated to ensure
transparency. While national and international consensus may not exist and may be slow to emerge, suggesting a
value for a BO and the associated rationale would be expected to make a valuable contribution to the process of
national and international consensus building.
Application of the ALARP concept by a technology developer
The flow chart in Fig. 2 exemplifies the procedure that a technology developer (designer) could follow
to confirm compliance of an NES under development with the INPRO methodology CR3.1 in the area of
environmental stressors (optimization of the measures to reduce environmental impact).
The quantity, optimized in an ALARP analysis, could be an individual stressor level, a weighted combination
of stressor levels (e.g. estimated dose) or some other risk related function of the system parameters. The values of
BL and BO are to be set on the basis of expert judgement, best technology available, regulatory clearance levels or
other information.
The depth that the ALARP analysis can reach depends on the maturity level of the NES assessed. For early
designs that are still at the conceptual stage, the optimization process could be guided by establishing a figure of
merit for acceptable limits and restrict the ALARP procedure to stressors (radioactive as well as non-radioactive,
if relevant) that have been identified as being the most significant or of the highest priority. Further reductions
of the number of stressors could be accepted only if they have been appropriately justified by the designer, with
evidence provided in the analysis report.
The ALARP concept might be applied to the whole NES under development, for which adequate BL/BO
values would need to be defined. In the case when the optimization occurs both at the individual facility (component)
level and at the whole system level, care needs to be taken to ensure that double counting does not occur. As an
example, in addition to a BL and a BO on 14C emitted from a reactor or a reprocessing facility, a BL and BO for the
aggregated 14C from the entire NES under development, including reprocessing and waste treatment facilities, can
also be set (see also the discussion below on the reduction of collective doses).
In the following, the proposed approach for each facility (component) is described in more detail, as it
corresponds to the way that ALARP is mostly applied in current practices.
If the analysis is performed in several stages for addressing successive levels of maturity of the NES being
developed or for considering possible changes in the regulations of the Member State or in international standards,
the BL and BO values might also need to be redefined, and the ALARP analysis may become more refined and
complex.
If the NES being developed is just at its conceptual stage, the ALARP demonstration may be reduced to a
discussion or a series of statements (illustrations) aimed at showing that modifications to the system that could
reduce these stressors have been considered, and that these stressors cannot practicably be reduced further. At the
very least, the developer (designer) should acquire evidence that sufficient thought has gone into evaluating the
potential of adjustments in the NES facilities and processes to reduce environmental effects. The descriptive
demonstration, adequately reported in the analysis report, should be finalized in the following phases of design
development. Reference[2] explains that the requirement for risks to be ALARP “is a requirement to take all
measures to reduce risk where doing so is reasonable. In most cases this is not done through an explicit comparison
of costs and benefits, but rather by applying established relevant good practice and standards.”
Hence, it is expected that if an analysis of more refined stages of the design process is performed, this should
verify ALARP for the same component(s)/stressor(s), and, if the design is changed, track the reasons for technical
modifications in relation to the previous ALARP analysis.
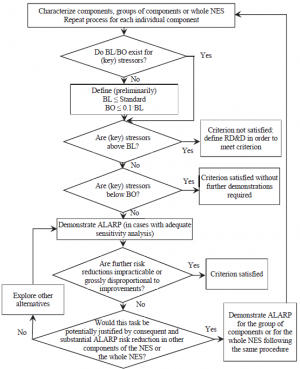
If a facility, or the whole NES, is at a more advanced state of development, ALARP may require the application
of tools for aiding in decisions such as CBA or MCDA[2][4]. It may happen that ALARP implementation in a
given facility may be challenging (stressors must be below BL for licensing) because of compensating effects across
the spectrum of stressors of the facility itself or group of facilities of the NES. Reference[2] notes that “where
there are several risks which interact, whether arising from a single hazard or from different connected hazards,
there may be a need for balancing to achieve the best overall solution.” In this case, the developer (designer) should
demonstrate and document that ALARP is fulfilled at a higher level by analysing groups of components or the
NES as a whole (see Fig. 2). A sensitivity analysis may be useful to show the robustness of the adopted technical
solution by varying key parameters (see the discussion below on the proportion factor between costs and risks).
CBA and/or MCDA need to be applied considering all aspects within the boundary of the assessment.
With reference to Fig. 2, what ‘gross disproportion between costs and risk reduction’ means for application,
no algorithm has been defined by the risk and safety authorities adopting ALARP, rather only a general guidance
for judgement to allow flexibility on a case by case basis. For current technology, ALARP involves consideration
of good practices[5]. However, this may change owing to innovation, which is improving the degree of control,
cost changes or knowledge about hazards[2]. For new technologies in an NES, evolving good practices could be
followed to start with, and then consideration given to whether there are alternative processes or procedures that
could be performed to reduce the risk on the basis of first principles to compare the risk with the sacrifice involved
in further reducing it. In many instances, such ‘first principles’ comparisons can be carried out qualitatively,
i.e. using common sense or exercising professional judgement or experience[5]. In the case when the costs are
definitively very high and the reduction in risk is just marginal, then ALARP is likely demonstrated, and no further
improvements need to be designed or implemented. Conversely, in most cases where the improvements identified
are simple or cheap to implement and the risk significantly reduced, these improvements are required, and ALARP
can be considered to be achieved without further analysis[5].
For less clear cut situations, which are most likely for innovative technologies, a more formal CBA may
provide additional insight to help to arrive at a judgement. In a CBA, both risk and sacrifice are converted into
monetary terms. In a standard CBA, the rule applies that the measure should be adopted if benefits outweigh costs.
However, in ALARP judgements, the measure has to be adopted unless the sacrifice is grossly disproportionate
to the risk. Therefore, “the costs can outweigh benefits and the measure could still be reasonably practicable to
introduce. How much costs can outweigh benefits before being judged grossly disproportionate depends on factors
such as how big the risk is to begin with (the larger the risk, the greater can be the disproportion between the cost
and risk)”[5].
Although individual doses to members of the critical groups are used for establishment of standards, hence
of BL and BO values, for the CBA, the total social costs of all emissions (radiological as well as non-radiological)
have to be taken into account. Therefore, it is the collective dose (see Appendix IV) that should be used. The scope
of the analysis depends on the choice by the developer (designer) of the consistency within the analysis. Ideally,
externalities should be evaluated considering the LCA of the entire cycle, thus including secondary burdens from
flows of material and energy into the system. However, in the case when the options to be addressed in the ALARP
procedure would not meaningfully change the secondary contributions in relative terms, the analysis can be
restricted to the effect of primary (or direct) burdens only. Studies of external costs for current nuclear technologies
can be found in Refs[6][7][8][9].
The ALARP process should also take into account risks that may affect future generations, as they may stem
from radioactive waste management and decommissioning. Reference[2] notes that “risks should be assessed in a
holistic manner and not restricted to part of the overall time period or part of a process.” Further elaboration points
out that: “Although it could be argued that the next few generations may gain some indirect benefit, the uncertainty
of how they will view the risks left to them (and indeed the uncertainty of any benefits further into the future)
argues for a precautionary approach and hence a particularly stringent demonstration that risks are indeed ALARP.”
Considering that the demonstration of long term sustainability for an NES is the ultimate objective of an INPRO
assessment, the requirement of consideration of effects into the future should be even stronger when applied to an
NES under development.
The following information, although referring to the ALARA approach, may also be of interest for ALARP.
The DOE recommends that for the purpose of comparing ALARA alternatives for operational systems, the lifetime
of the facility generally is a basis for truncating collective dose estimates, temporally[4]. However, where
cleanup, restoration and waste management activities are necessary, the time frame for the analysis can be much
longer. In particular, for long lived radionuclides, integration times may be determined by the uncertainties in
scenarios and by the physical parameters affecting dose rates. The DOE states that, typically, it is only appropriate
to conduct quantitative comparisons for a few hundred years or less. Although evaluating doses for periods of
up to a thousand years may provide useful information, periods beyond this length of time should not be used in
quantitative ALARA assessments[4]. However, although this position may be adopted for an analysis, provided
it is justified by the nature and amounts of the releases, consideration of longer integration times may be useful in
other parts of the analysis.
Reference[2] defines that: “The safety benefit of the improvement is the incremental difference in risk
between the two estimates in terms of the detriments (i.e. all the adverse consequences) and their likelihoods,
summed over the remaining life of the facility.” This includes detriments from normal operation such as radiation
exposures of workers and the public; detriments from accidents which again includes radiation exposures to
the public and workers but also includes other detriments such as the need to decontaminate areas, evacuation,
relocation and food restrictions. Valuation of detriments is a subject of much discussion and research[2].
The UK HSE[10] uses £1.5 million (2009) for the value of preventing a statistical fatality. In the case of
death caused by cancer, the HSE takes the view that people are prepared to pay a premium. Thus, a higher value,
such as twice that defined above, should be used. It is suggested that this higher value is also appropriate for all
radiation deaths.
In the European study ExternE[11][9], the value for average social (or external) costs in Europe attributed
to any cancer, whatever its origin and outcome (fatal or non-fatal), is €2 million. In the case where an assessment is
performed for a different country, if ‘willingness to pay’ (WTP) estimates are lacking, values established in Europe
or the United States of America can be adjusted to specific conditions using the ratio of the purchasing power
parity gross national product per capita. The adjustments are based on the assumption that there is an ‘elasticity’ of
WTP with respect to real income. An example of the application of this approach to the assessment of electricity
scenarios (including nuclear) for the Shandong Province, in China, can be found in Ref.[12].
Use of external cost calculations implies verification, to the latest available information on damage
functions and external costs in publications, and detailed reporting on the assumptions/options used or possible
modifications/adaptations.
The following applies to ALARA as implemented in the United States of America. However, the information
may also be useful for ALARP assessments. For the costs associated with units of doses, the linear relationship of
health effects with dose is postulated, thus making the monetary value independent of the magnitude of individual
doses. Several rationales have been adopted for the estimation of the monetary value of a unit of collective dose or
other effects of non-radioactive substances, which can be found in the literature. The use of WTP, i.e. the willingness
to commit resources to avoid the negative impact, is considered here. As an example of application, in 1995, the
NRC[13]] re-evaluated the monetary equivalent value and issued regulatory analysis guidelines that recommend
US $2000/man rem (US $200 000/Sv) as the current monetary equivalent value for converting collective dose
to US dollars[4]. The DOE accepted the NRC recommendation, and, because of the uncertainty in the values
used in ALARA analyses, further elaborated that detailed ALARA evaluations use the range for comparing
alternatives. The DOE evaluations to support ALARA analyses apply monetary equivalents for a man rem in the
range US $1000–6000 with a nominal value of US $2000[4][14]. Assuming that one man rem corresponds to
a potential risk of 5 × 10−4 radiation induced fatal health effects (generally cancers) for the population, the range
recommended by the DOE would correspond to a range of US $2 million to US $12 million per hypothetical
radiation induced cancer death averted[4].
In cases where, in addition to health (and environmental) risks and costs of measures to reduce them, several
other non-health detriments are identified, these should also be included in the analysis with societal costs when
appropriate and feasible. These effects can be physical (e.g. waste heat discharge, noise or damage from potential,
very low frequency/high consequence accidents — for the latter, see Ref.[9]) or sociopolitical (e.g. aversion,
opposition or proliferation). Some may be quantified in monetary terms and could be included in the CBA.
However, in most cases, such quantification has strong subjective elements, i.e. weighting factors may intervene
(see discussion in Ref.[4], p. 18). Therefore, in cases where non-monetary aspects are identified or anticipated as
a crucial part of the decision on which measures identified in an ALARP process should be implemented, a formal
MCDA may be best applied.
As mentioned in Ref.[2], if the risks (given by frequency multiplied by the consequences) are high, then the
demonstration of ALARP should be more rigorous than if the risk is low. Where risks are high, the consequences
should be weighted more than the frequency, and the ALARP demonstration should be accurate.
The technical assessment guide of the Office for Nuclear Regulation[2] provides specific guidance for
inspectors of nuclear installations on what they should expect of a licensee in meeting legal requirements to reduce
risks to ALARP. It suggests, on the basis of previous experience, a disproportion factor between costs and risk
reduction benefits of up to three for workers, whereas for risks to the public, the factor would depend on the level
of risk, suggesting a factor of about two for low risks and about ten for higher risks. These factors may be applied
in an analysis, unless other rationale are provided.
Owing to the uncertainties at various levels in the system under scrutiny and in the methods used for the
prediction of releases and associated consequences, it is suggested in Ref.[2] to base the judgement on the
appropriateness of the proportion factor on the robustness of the analysis. This factor should also be one of the
parameters to be varied in a sensitivity analysis
.
Considering that, usually, the scope of an environmental analysis includes also anticipated operational
occurrences, it is useful that the National Radiological Protection Board (NRPB) has produced guidance relating
to the dose saved resulting from routine exposure or minor incidents, i.e. stochastic effects, up to about 100 mSv.
The NRPB recommends that an increasing multiplier is used in ALARP demonstrations as the dose increases,
and also recommends the HSE Nuclear Safety Directorate to assess licensees’ submissions using this approach.
Reference[2] suggests that a factor (for costs outweighing risks) of less than ten in the vicinity of the intolerable
region is unlikely to be acceptable and, for hazards that can cause large consequences, the factor may need to be
larger still. This requirement may be understood as being even more pronounced for an NES under development,
especially at the early design stages for which the uncertainties may be predominant on the cost differences
calculated for risk reduction measures. The recommendation of Ref.[2], discussed above, to include the proportion
factor within the parameters to be varied in a sensitivity analysis is repeated here.
Example of application to a reprocessing facility
One of the rather few examples of the application of ALARP to a facility of a current NES[15] reports on the
Environment Agency’s re-examination of the Sellafield reprocessing plant’s radioactive discharge authorizations
from 2000 to 2002. This example would have the potential to also serve for assessing the ALARP concept of future
reprocessing plants. However, the information available in Ref.[15] is rather limited, and is therefore insufficient
for full understanding of the procedure used (e.g. missing information on risk levels before implementation of
ALARP, how the optimized actions were justified and on the costs involved).
Table 1 presents the doses to the critical groups caused by radioactive discharges from the Sellafield
reprocessing plant in 2001[15].
Aerial effluent discharges | Liquid effluent discharges | ||
---|---|---|---|
Radionuiclide | Critical group dose (adult) (μSv) | Radionuclide | Critical group dose (seafood consumer) (μSv) |
Ar-41 | 31 | Tc-99 | 23 |
Sr-90 | 11 | C-14 | 5 |
Ru-106 | 3 | Cs-137 | 4 |
Cs-137 | 3 | Ru-106 | 2 |
Pu alpha | 2 | Sr-90 | 1 |
C-14 | 1 | Co-60 | 1 |
Kr-85 | 1 | Others | 1 |
I-129 | 1 | ||
Am-241 | 1 | Am-241* | 50 |
I-131 | 1 | Pu alpha* | 28 |
Others | 3 | Pu-241* | 4 |
Total | 58 | Total | 119 |
* - Historic contribution from discharges prior to the Site IonExchange Effluent Plant (SIXEP) and the Enhanced Actinide Removal Plant (EARP) have been introduced.
The strategy of the operator, British Nuclear Fuels (BNFL), for controlling radioactive discharges from the
Sellafield site consists of:
(a) Designing plants to minimize radioactive arisings, and subsequent discharges and disposals, where practicable
by:
- Employing processes that avoid the production of gaseous and liquid by-products, and that avoid solid waste generation (it is a general rule that because environmental impacts are greatest per unit activity for aerial releases, and are smallest for solid wastes, the design of the process reflects this hierarchy for the avoidance of generation of by-products).
- Proper design of abatement systems.
- Maximizing dispersion.
(b) Commissioning, operating and decommissioning plants, minimizing liquid and aerial discharges by means of:
- Prioritization in terms of dose impacts on the public. “To ensure resources are used in the most cost-effective manner, BNFL concentrates its ongoing research and development work on reducing those discharges which result in the highest doses or highest chemical toxicity, focusing effort on those areas in which there is the greatest potential for minimising environmental impact. For radioactive substances, it is generally the policy to use critical group dose as the key determining factor, though consideration is also given to collective doses”[15]. Table 1 shows that as of 2001 discharges, only a few radionuclides make almost all doses to the critical groups; this helps to identify where to intervene first in order to reduce risks to the population. Incidentally, the total critical group doses are within the BO–BL range of 0.02–1 mSv, as requested by the HSE.
- Increasing the magnitude of operations by decreasing the magnitude of wastes generated for eventual disposal.
- Addressing specific airborne emitted species: decreasing discharges of 41Ar, 14C and 129I.
- Addressing specific water-borne emitted species: abating 137Cs, 60Co, 239Pu and 99Tc.
(c) Developing new technologies.
(d) Implementing appropriate management systems.
Best available techniques
Concepts
According to the Convention for the Protection of the Marine Environment of the North-East Atlantic[16]: “The term ‘best available techniques’ means the latest stage of development (state of the art) of processes, of facilities or of methods of operation which indicate the practical suitability of a particular measure for limiting discharges, emissions and waste. In determining whether a set of processes, facilities and methods of operation constitute the best available techniques in general or individual cases, special consideration shall be
given to:
(a) comparable processes, facilities or methods of operation which have recently been successfully tried out;
(b) technological advances and changes in scientific knowledge and understanding;
(c) the economic feasibility of such techniques;
(d) time limits for installation in both new and existing plants;
(e) the nature and volume of the discharges and emissions concerned.”
Related to the application of BAT is the application of BEP, which is defined in the Convention[16] as “the application of the most appropriate combination of environmental control measures and strategies”. The Convention further states that[16]:
“In determining what combination of measures constitute best environmental practice, in general or individual cases, particular consideration should be given to:
(a) the environmental hazard of the product and its production, use and ultimate disposal;
(b) the substitution by less polluting activities or substances;
(c) the scale of use;
(d) the potential environmental benefit or penalty of substitute materials or activities;
(e) advances and changes in scientific knowledge and understanding;
(f) time limits for implementation;
(g) social and economic implications.”
In addition, BEP for a particular source will change with time “in the light of technological advances, economic and social factors, as well as changes in scientific knowledge and understanding”[16]. Finally[16]:
“If the reduction of inputs resulting from the use of best environmental practice does not lead to environmentally acceptable results, additional measures have to be applied and best environmental practice redefined.”
Regardless of the approach that is used for determining the optimal option, it should be recognized that
judgements are required about the relative significance of the factors involved, which includes dialogue between
the regulatory body and the operator, as well as other interested parties, particularly those who might be affected by
the situation, facility or planned activity (e.g. local community representatives).
Formal decision aiding techniques may be used in the optimization process, especially for nuclear fuel cycle
facilities. However, when the doses to the representative person are assessed to be very low (e.g. of the order of
10 μSv/a or less), a formal analysis of the optimization of protection is generally not necessary. The magnitude
of exposure, the number of exposed individuals and the likelihood of exposure should be assessed for each of the
options being considered. For complex facilities, elements of the radiological assessments should be made available
at an early stage, and the development of the assessment may be an iterative process involving discussions between
the operator, the regulatory body and other interested parties. The assessment should be refined as the development
of the facility moves towards the stage at which an application for a licence for the planned facility is to be made
(e.g. a licence to operate).
Application of best available techniques in environmental impact assessment
BAT assessment is typically undertaken when making decisions regarding the development and
implementation of appropriate discharge standards to ensure environmental protection. This can include situations
when standards for the discharge of radioactive material are being developed, for planning the management and
disposal of radioactive waste, when authorizations are undergoing a significant amendment or when facilities are in
a sensitive environment.
An example is provided here for the Gunnar Mine Site, which is an abandoned uranium mine and mill site
in northern Saskatchewan, Canada. The site was operated between the late 1950s and early 1960s under a less
stringent regulatory regime than today. Uranium ore was initially mined from an open pit from 1955 to 1961, and
later, from an underground operation between 1957 and 1963. The Gunnar Mine Site officially closed in 1964, with
little or no remediation of its facilities.
A stepwise decision tree approach was developed and applied to identify all potentially feasible remedial
options, as part of the EIA process, and to map out key decision points during the licensing phase of the project,
when final remedial options were selected from the list of options. Although the example provides an illustration of
the approach for the remediation of the Gunnar Mine Site, the same approach can be used for optimization of the
options intended for environmental protection.
A number of end points (or desired outcomes of the remediation efforts) were identified for the site, as
follows:
- The site does not pose unreasonable public health or environmental risks.
- Flora and fauna adjacent to the site are not significantly affected by contaminants.
- Traditional use of resources adjacent to the site can be safely conducted.
- The desire is to have the site managed through an institutional controls programme for long term care and maintenance.
Screening framework for evaluation of remedial options
In conducting the alternatives assessment, all available options were identified and evaluated, including all
aspects of each mine waste disposal alternative throughout the project life cycle, and all aspects of the project
(direct or indirect) that may contribute to the predicted impacts associated with each potential option[17][18].
The assessment considered the predicted quality and quantity of releases to the environment, and the corresponding
predicted effects on the environment. The assessment of alternatives also considered the full cost of each remedial
option throughout the project life cycle and its associated benefits, as an independent step from the technical review
of options.
Assessment of the predicted quality and quantity of releases to the environment, and corresponding effects to
the environment, are addressed through characterization of contaminant concentrations and key contaminant flow
pathways, and subsequently inputting data into the human health and ecological risk assessment (HHERA).
Evaluation of remedial options was conducted using a multitiered assessment approach based on
environmental and technical CR, utilizing the framework for assessment of alternatives proposed in Ref.[17], and
which is presented in Table 2.
Step | Definition | Criteria | Analysis | Deliverable |
---|---|---|---|---|
1 | Identify candidate alternatives | Threshold criteria | Mine waste disposal technologies
Mine waste disposal storage options Mine waste disposal sites |
Summary table
Location maps |
2 | Prescreening assessment | Prescreening criteria | Prescreening evaluation | Summary table |
3 | Alternative characterization | Characterization criteria (accounts) | Environmental criteria
Technical criteria Project economic criteria Socioeconomic criteria |
Summary tables |
4 | Multiple accounts ledger | Evaluation criteria (subaccounts)
Measurement criteria (indicators) |
Qualitative value scales | Summary tables |
5 | Value based decision process | Scoring
Weighting |
Quantitative analysis | Summary tables |
6 | Sensitivity analysis | Reconsider weighting | Quantitative analyses | Summary tables |
7 | Document results | Final report |
The summary of the major steps of the assessment provided in Ref.[18] specifies that these steps include:
- Development of a list of all possible candidate remedial options, with the input from stakeholders and without prior judgements;
- Screening of options to reduce the number and to provide assurance that any of the remaining options could prove to be the preferred option;
- Characterization of the remaining alternatives to ensure proper consideration of every aspect and nuance and the comparison of alternatives in a clear and concise format, to ensure complete transparency of the decision process;
- Identification of evaluation criteria that are linked to an effect and that easily differentiate the alternatives;
- Inclusion of quantitative value judgement in the decision process by scoring and weighting all evaluation criteria under the environmental, technical, economic and socioeconomic characteristics of each alternative;
- An assessment of the sensitivity of the decision making process.
An assessment of candidate Gunnar remedial options was conducted in general accordance with the framework for MCDA provided by Ref.[17], which is provided as a case study.
Remedial options analysis
A description of the key steps in remedial options analysis (see Table 2) was developed by Environment
Canada in 2011[17]. This approach has been applied to evaluate unfiltered candidate options for remediation at
the Gunnar Mine Site.
Step 1: Identification of candidate alternatives
A first step in the assessment of remedial options is to develop a list of all possible candidate remedial
options, with input from stakeholders and without prejudgement, i.e. to generate an ‘unfiltered’ list of remedial
options[17][18]. In doing so, a basic set of ‘threshold criteria’ (or ‘exclusion criteria’) may be established that
can then be used in the prescreening of unfiltered options, as part of step 2 of the remedial options analysis.
For the purposes of options assessment, candidate alternatives were identified using a two phased approach:
compilation of an unfiltered list of remedial options and then the evaluation of this list. The unfiltered list of
remedial options that was generated during the first phase was distributed for review and input to responsible
authorities, including provincial and federal regulatory and project partner stakeholder groups, in the second phase.
The unfiltered remediation alternatives were generated to address each of the following Gunnar Site components,
which were identified in the Gunnar EIA as the major ‘aspects’ of the site remediation:
- Waste disposal (i.e. disposal of waste generated by the demolition of former site infrastructure);
- Waste rock deposit;
- Open pit;
- Hydrocarbon contaminated soil;
- Waste rock seepage;
- Groundwater seepage;
- Gunnar main tailings (including tailings downstream of the dyke at the eastern boundary of the Gunnar main tailings deposit);
- Gunnar central tailings;
- Langley Bay/Back Bay (including tailings and aquatic receiving environment).
The candidate alternatives that were generated through this exercise constituted a broad list of waste disposal
technologies, storage options and disposal locations, both on-site and off-site.
Step 2: Prescreening assessment
Following identification of the candidate remediation alternatives (as part of step 1 above), a prescreening
assessment of the unfiltered list was conducted, based on threshold criteria (or exclusion criteria). The objective of
the prescreening was to optimize the decision making process, with focus on an appropriate and manageable set of
sufficiently detailed alternatives, as opposed to evaluating options that have obvious deficiencies[17].
Once the generic alternatives had been identified, based on reason, practicality and precedence, any ‘fatal
flaw’ for each alternative was identified via preliminary screening. The screening of options was performed against
threshold/exclusion criteria specific to the project objectives/desired end points. These criteria were established so
as to generate a simple ‘yes’ or ‘no’ answer, dictating which alternatives were to be carried through to more detailed
analysis. The established threshold (or exclusion) criteria were as follows:
- Does the alternative allow for compliance with a total external gamma dose criteria (mean value of ≤ 1 μSv/habove the background at a 1 m height, averaged over a 100 m × 100 m area and maximum value of 2.5 μSv/habove the background at a 1 m height, based on close out criteria for Cluff Lake, a uranium mine/mill sitein northern Saskatchewan further along in the decommissioning/remediation process than Gunnar[19])(Gunnar background = 0.7 μSv/h)? (Answer of ‘no’ indicates a fatal flaw.)
- Does the alternative allow for long term compliance with site specific/Saskatchewan Environment/Canadian Council of Ministers of the Environment guidelines/objectives/standards for soil and surface water quality? (Answer of ‘no’ indicates a fatal flaw.)
- Does the alternative allow for compliance with pertinent provincial/federal legislation? (Answer of ‘no’ indicates a fatal flaw.)
- Is the alternative a proven technology/has it been completed elsewhere with successful results? (Answer of ‘no’ indicates a fatal flaw.)
- Does the alternative improve the level of risk at the site? (Answer of ‘no’ indicates a fatal flaw due to an increase in risk.)
- Is there a potential risk of catastrophic failure? (Answer of ‘yes’ indicates a fatal flaw.)
- For waste disposal, is the proposed disposal site sufficiently large to accommodate waste? (Answer of ‘no’ indicates a fatal flaw.)
Each alternative receiving a positive evaluation (i.e. with no fatal flaws identified) was carried through a
multiple accounts analysis (as demonstrated in Table 2), where the impacts from each alternative were assessed
through a transparent scoring and weighting process.
Step 3: Characterization of alternatives
Once the prescreening assessment of candidate remedial alternatives had been completed, the remaining
options required characterization. During options characterization, the options for each major site element were
evaluated by a multidisciplinary project team consisting of individuals with expertise in the natural sciences,
contaminant dynamics and engineering. Only potentially feasible alternatives were carried through to this stage
of analysis. Feasible alternatives included those that could represent implementable remedial options, with no
fatal flaws being identified during prescreening with respect to project objectives and end points. For clarity and
differentiation from prescreening of remedial ‘alternatives’, potentially feasible alternatives that were carried
through to detailed analysis were renamed as ‘options’ (as opposed to alternatives).
The outcomes of the evaluations of HHERA were derived in the context of the following broad evaluation
criteria, which were developed for the site as part of the Gunnar EIA:
- Human health risks;
- Terrestrial wildlife and vegetation;
- Aquatic life and vegetation.
Within each of the above broad criteria, more detailed evaluation criteria (or ‘subaccounts’) were established,
as described under step 4 below.
The focus of the evaluation of options with respect to HHERA was centred around potential option related
chemical and radiological impacts to members of the public and the environment. In addition, physical impacts
to terrestrial and aquatic life and vegetation were considered as part of the ecological risk assessment related
evaluation criteria. As the Gunnar Mine Site has been secured and is not open to the public without orientation, and
work activities are being planned and implemented to protect people, physical impacts to humans were considered
as part of the evaluation of constructability.
The constructability evaluation was focused on the following two evaluation criteria:
- Potential risks to humans (i.e. workers) and the environment during the execution of the remediation (as part of the ‘active remediation phase’);
- Constructability/feasibility/efficacy of a given remedial option from a ‘practicability of execution’ perspective.
Following evaluation of remedial options with respect to HHERA and constructability, further screening was
conducted to identify the potentially acceptable options based on public preference, gained through engagement
of local communities and leaders, both through providing residents with information on the project, and receiving
feedback and advice from communities. This was accomplished through community meetings, surveys and
other forums, which provided important insight with respect to public preferences regarding the planning and
implementation of the project, and which were considered in the evaluation of the Gunnar remedial options.
Step 4: Multiple accounts ledger
Following identification and characterization of alternatives in steps 1–3, it was necessary to then evaluate the
options that were identified as potentially feasible, by identifying elements that could be used to differentiate them.
This was accomplished through the development of a multiple accounts ledger, which consisted of the following
two elements: subaccounts (also called evaluation criteria) and indicators (also called measurement criteria).
Subaccounts (or evaluation criteria) were developed using the characterization criteria that had been selected
during step 3. Unlike the characterization criteria, which were factual criteria that had been assigned with no a
priori judgement being made regarding any of the alternatives under consideration, the evaluation criteria were
developed with consideration of the material impact (benefit or loss) associated with each of the options under
evaluation.
Evaluation criteria with respect to the Gunnar remediation project were, therefore, set in the context of three
broad categories (or ‘accounts’): HHERA, constructability and public preference, as described above.
Within the above evaluation criteria, a number of qualitative and quantitative measurement criteria were
established, which were then scored to identify potentially feasible remedial options.
The following measurement criteria were identified with respect to potential worker and ecological health
risks during remediation:
- Physical hazards (safety risks);
- Worker health risks via exposure to non-radiological and radiological contaminants (soil or dust inhalation, dermal contact), ambient radon exposure and external radiation exposure;
- Risks to terrestrial wildlife generated;
- Risks to aquatic life generated.
The following measurement criteria were identified with respect to constructability, feasibility and efficacy during remediation:
- Estimated construction duration;
- Cost;
- Ability to mitigate pertinent exposure pathways;
- Regulatory acceptance;
- Long term waste generation;
- Requirement for control, monitoring and/or maintenance (i.e. institutional control).
The possible Gunnar remedial options were evaluated in the context of a single integrated measurement
criterion that has been identified as ‘public preference’, which was scored as described in further detail below.
Step 5: Value based decision process
Once a multiple accounts ledger had been created, with criteria to facilitate the evaluation and measurement
of possible remedial options, it was necessary to score and weight all the indicators, subaccounts and accounts,
such that merit ratings for each option could be quantitatively determined. In doing so, the following processes
were undertaken:
- Scoring;
- Weighting;
- Quantitative analysis.
Scoring of the possible Gunnar remedial options from the perspective of HHERA in the long term was conducted using both quantitative and qualitative measurement criteria. Quantitative criteria were based on the concentrations of contaminants released to the environment in the long term for a given option relative to environmental quality guidelines, whereas qualitative criteria provided a relative comparison of remedial options in cases for which no quantitative guidelines existed against which measurement criteria could be compared. On this basis, the following scoring criteria were developed:
- A value of 0 indicates exceedance of the guideline by 100-fold or greater/physical risk that exceeds the baseline risk; this option performs very poorly against listed criteria.
- A value of 1 indicates exceedance of the guideline by 10- to 100-fold/physical risk that is similar to the baseline risk; this option is the same or has a very small improvement compared with the existing situation.
- A value of 2 indicates exceedance of the guideline by 1- to 10-fold/physical risk that is lower than the baseline risk; this option has a moderate improvement compared with the existing situation.
- A value of 3 indicates not applicable; this option has no exceedance of guideline values or a large improvement compared with the existing situation/best performing option.
To differentiate the performance of the options versus the prescribed criteria, numerical rankings were assigned as a quantitative indicator of impact. The following measurement scheme was used with respect to constructability:
- A value of 0 indicates the option performs very poorly against listed criteria.
- A value of 1 indicates the same or a very small improvement compared with the existing situation.
- A value of 2 indicates moderate improvement compared with the existing situation.
- A value of 3 indicates not applicable/large improvement compared with the existing situation/best performing option.
In terms of options that were ‘do nothing’ or very near to do nothing, many of the criteria in the technical
analysis were evaluated as ‘not applicable’. These not applicable designations were given a ranking of 3, to indicate
a high ranking in terms of subaccounts, such as duration or cost, in comparison with options for which some action
is taken.
Scoring of each remedial option with respect to public preference was undertaken using an integrated approach
to incorporate information that had been received from community members and leaders through a diversity of
consultation approaches. The outcomes of such approaches, which included community meetings, flyers, surveys
and many others, were used to inform the scoring in the context of the following scoring criteria:
- A value of 0 indicates this option is unacceptable or that local communities are opposed to the option.
- A value of 1 indicates local communities would consider it as a possible option, but with reservations.
- A value of 2 indicates local communities would consider it as a possible option.
- A value of 3 indicates local communities would consider it as a preferred option.
It should be noted that in assigning scores of 1 versus 2 above, values were set to distinguish options that
were considered possible by local communities, in one case, with reservations, and in the second case, with few
to no reservations. For example, a score of 1 was assigned for options involving transport of hazardous materials
across Lake Athabasca. In such scenarios, it was necessary for activities to be conducted in close consultation with
communities owing to the value that northern communities place on water quality and the concerns of catastrophic
releases of hazardous materials to Lake Athabasca. Despite these reservations, it was possible to transport hazardous
materials across the winter ice road, working closely with local communities.
As part of the analysis, weighting factors were applied to the raw scores that had been generated during the
evaluation of possible Gunnar remedial options. In this way, it was possible to assign a relative importance of a
given measurement criterion compared to others.
As a preliminary step, weighting factors were assigned to each of the three accounts (HHERA, constructability
and public preference), as follows:
- A value of 40% for HHERA;
- A value of 40% for constructability;
- A value 20% for public preference.
Within each of the above three accounts, individual measurement criteria were also weighted and justified to
reflect their relative importance.
Once scoring and weighting of each possible remedial option was completed, it was necessary to conduct a
quantitative analysis of the information. This involved compiling the indicator value S (‘score’) for each indicator
(or measurement criterion), along with the weighting factor W, and calculating the product of these to generate a
combined indicator merit score (S·W) for each possible remedial option[17].
Based on these data, it was possible to take the sum of the indicator merit scores for each evaluation criterion
(or subaccount) to generate a subaccount merit score for each, i.e. Σ{S·W}. Within a given subaccount (or evaluation
criterion), the resultant subaccount merit scores could then be directly compared across possible remedial options.
To facilitate further comparison of each subaccount merit score against values for other subaccounts, the
scores were normalized to the same point scale used to score each indicator value. In doing so, the subaccount
merit score was divided by the sum of the weightings (Σ W) to yield a subaccount merit rating[17]:
The same procedure can be undertaken to weight and normalize scoring for accounts (in this case, HHERA,
constructability and public preference) to generate account merit scores (Σ{Rs·W}) and account merit ratings
(Σ{Rs·W}/ΣW) for each remedial option.
As a final step, option merit scores (Σ{Ra·W}) and option merit ratings (Σ{Ra·W}/ΣW) can be determined for
each possible Gunnar remedial option.
It should be noted that even with the multiple layers of analysis, it is possible for certain options to score
identically, against all criteria, for a certain aspect of the site. In the event of such scoring/ranking, the performance
of the option needs to be measured against the total available score, as described below.
Step 6: Sensitivity analysis
Any decision making process will be inherently subject to some degree of bias and subjectivity. Therefore,
it is important that a framework is followed to ensure that any decisions arising from the options screening and
selection process are transparent and reproducible by external reviewers[17]. With this in mind, the options
analysis was completed for the Gunnar Mine Site in multiple tables, rating the potential remedial options separately
with respect to performance against: (i) HHERA, (ii) constructability and (iii) public preference, to allow for such
transparency.
A sensitivity analysis can involve a very large number of permutations and combinations, which in itself can
impose bias and subjectivity. Therefore, for the purposes of the Gunnar evaluation, the sensitivity analysis was
kept simple, with the ultimate goal of evaluating the impact of the respective indicator weights (W) on the selection
of potentially feasible remedial options, based on the initial ranking (i.e. assignment of a value of 0–3 for each
measurement criterion and remedial option combination). As such, the analysis was conducted by simply setting all
weightings to a value of 1, effectively removing the value bias between one measurement criterion and another. In
this case, remedial options achieving relatively higher sums of indicator values measured against the measurement
criterion values may be more feasible for a given site aspect than those with lower indicator values. A sensitivity
analysis was conducted to evaluate this.
Summary of the study results
An assessment of candidate remedial options was conducted in general accordance with the framework for
MCDA provided by Ref.[17]. The analysis consisted of multiple stages of analysis, as demonstrated in Table 2.
Based on this approach, a comprehensive, unfiltered list of possible remedial options for the Gunnar Mine Site was
evaluated and scored to identify potentially feasible remedial options.
For the purposes of this analysis, a potentially preferable option was identified as the option for which the
highest option merit rating was determined. Owing to the inherent complexity of the Gunnar remediation project,
it was necessary to subdivide remedial options into a number of interconnected site aspects (e.g. submerged and
unsubmerged unconfined tailings, waste rock piles, open pit, etc.), which were individually scored as separate
matrices, with respect to HHERA and public preference.
Based on this analysis, potentially preferable remedial options were identified to address each Gunnar Site
aspect; however, due to the lack of historical records and monitoring data, it was not possible to identify a single,
‘preferred’ remedial option. Instead, potential remedial options could be subdivided into three categories[20]:
(a) Options that are relatively straightforward to implement, with little uncertainty, e.g. covering unconfined
tailings, water diversions, removal of contaminated debris from waste rock piles and addressing physical
hazards associated with unstable slopes on the waste rock piles.
(b) Options that require additional monitoring data and follow-up assessment prior to selection of a final
remediation approach, e.g. further surface water and hydrogeological monitoring to determine contaminant
transport pathways between sources and receiving environments.
(c) Options that are dependent upon the selection of remedial approaches for other site aspects that have to be
determined before the first one can proceed, e.g. decisions on the pit cannot be determined until it has been
decided if the waste rock will be placed in the pit or covered and left in place.
See Also
[ + ] Assessment Methodology | |||||
---|---|---|---|---|---|
|
References
- ↑ INTERNATIONAL ATOMIC ENERGY AGENCY, Methodology for the Assessment of Innovative Nuclear Reactors and Fuel Cycles, Report of Phase 1B (first part) of the International Project on Innovative Nuclear Reactors and Fuel Cycles (INPRO), IAEA-TECDOC-1434, IAEA, Vienna (2004).
- ↑ 2.00 2.01 2.02 2.03 2.04 2.05 2.06 2.07 2.08 2.09 2.10 2.11 OFFICE FOR NUCLEAR REGULATION, Guidance on the Demonstration of ALARP (As Low As Reasonably Practicable), Nuclear Safety Technical Assessment Guide No. NS-TAST-GD-005, Rev. 7, ONR, Bootle (2015).
- ↑ INTERNATIONAL ATOMIC ENERGY AGENCY, Guidance for the Application of an Assessment Methodology for Innovative Nuclear Energy Systems, INPRO Manual: Environment, Vol. 7, Final Report of Phase 1 of the International Project on Innovative Nuclear Reactors and Fuel Cycles (INPRO), IAEA-TECDOC-1575/Rev. 1, IAEA, Vienna (2008).
- ↑ 4.0 4.1 4.2 4.3 4.4 4.5 4.6 UNITED STATES DEPARTMENT OF ENERGY, Applying the ALARA Process for Radiation Protection of the Public and Environmental Compliance with 10 CFR Part 834 and DOE 5400.5 ALARA Program Requirements, Vol. 1, Discussion, USDOE, Washington, DC (1997).
- ↑ 5.0 5.1 5.2 5.3 HEALTH AND SAFETY EXECUTIVE, ALARP Suite of Guidance, [1]
- ↑ EUROPEAN COMMISSION, ExternE, Externalities of Energy, Vol. 10: National Implementation, Rep. EUR 18528, Office for Official Publications of the European Communities, Luxembourg (1999).
- ↑ DONES, R., GANTNER, U., HIRSCHBERG, S., DOKA, G., KNOEPFEL, I., Environmental Inventories for Future Electricity Supply Systems for Switzerland, Rep. No. 96 07, Paul Scherrer Institut, Villigen, Swiss Centre for Life Cycle Inventories, Dübendorf (1996).
- ↑ DONES, R., et al., New Energy Technologies, Final Report on Work Package 6, Release 2, ExternE-Pol Project ‘Externalities of Energy: Extension of Accounting Framework and Policy Applications’, European Commission, Brussels (2005).
- ↑ 9.0 9.1 9.2 EUROPEAN COMMISSION, New Elements for the Assessment of External Costs from Energy Technologies, European Commission, Brussels (2004).
- ↑ HEALTH AND SAFETY EXECUTIVE, Reducing Risks, Protecting People: HSE’s Decision-Making Process, Crown, Colegate (2001).
- ↑ EUROPEAN COMMISSION, ExternE, Externalities of Energy, Methodology 2005 Update, Rep. EUR 21951, Office for Official Publications of the European Communities, Luxembourg (2005).
- ↑ HIRSCHBERG, S., et al., Environmental Impact and External Cost Assessment, Vol. 4 in Integrated Assessment of Sustainable Energy Systems in China: The China Energy Technology Program, Alliance for Global Sustainability Series, Kluwer Academic Publishers, Dordrecht (2003).
- ↑ NUCLEAR REGULATORY COMMISSION, Reassessment of NRC’s Dollar per Person-rem Conversion Factor Policy, Rep. NUREG-1530, NRC, Washington, DC (1995).
- ↑ UNITED STATES DEPARTMENT OF ENERGY, Estimating Costs for Man-rem Exposures, US DOE Office of Waste Management, M.H. Chew & Associates, Inc. (1996).
- ↑ 15.0 15.1 15.2 15.3 15.4 MORLEY, B., Best practicable means (BPM) and as low as reasonably practicable (ALARP) in action at Sellafield, J. Radiol. Prot. 24 1 (2004) 29.
- ↑ 16.0 16.1 16.2 16.3 16.4 OSPAR COMMISSION, The Convention for the Protection of the Marine Environment of the North-East Atlantic, Paris (1992).
- ↑ 17.00 17.01 17.02 17.03 17.04 17.05 17.06 17.07 17.08 17.09 17.10 ENVIRONMENT CANADA, Guidelines for the Assessment of Alternatives for Mine Waste Disposal, Environment Canada (2011).
- ↑ 18.0 18.1 18.2 CANADIAN NUCLEAR SAFETY COMMISSION, Management of Uranium Mine Waste Rock and Mill Tailings, Rep. RD/GD-370, CNSC, Ontario (2012).
- ↑ CANADIAN NUCLEAR SAFETY COMMISSION, Comprehensive Study Report: Cluff Lake Decommissioning Project, CNSC, Ontario (2003).
- ↑ YANKOVICH, T., HACHKOWSKI, A., KLYASHTORIN, A., “Options evaluation for remediation of the Gunnar Site using a decision-tree approach” (Proc. Int. Conf. Radioecology and Environmental Radioactivity, Barcelona, 2014).